The purpose of this chapter is to explore the impact of Engineering Research Centers on colleges of engineering and engineering departments in terms of their research and education cultures, as well as the characteristics of engineering graduates who have had an ERC experience. The main issue to be explored is: Has the ERC culture of interdisciplinary engineered systems research in collaboration with industry produced sustained impacts on academic engineering where ERCs were funded?
10-A ERCs by the Numbers
This section provides information on the numbers of centers funded, their locations, and their lead and partner institutions in order to position the impacts noted below in the context of U.S. academic engineering.
10-A(a) Centers Funded
i. Numbers of Centers
The first six ERCs were awarded in March 1985. The original NAE Guidelines for Engineering Research Centers[1] recommended that the Program should build to a total of 25 centers, an ambitious number at that time. As Figure 10-1 depicts, there were three generations of ERCs funded between 1985 and 2017. Gen 1 ERCs (1985-1990) were the proving ground for the ERC construct and 21 ERCs were funded. Gen-2 ERCs (28 new ERCs and 3 EERCs) rested on the core ERC construct but with strengthened strategic planning and proof-of concept systems testbeds and increased outreach for broader impact and diversity. Between 2008 and 2017, 19 new Gen-3 ERCs with enhanced innovation programs were funded. By that time a total of 71 ERCs and EERCs had been funded. The number of centers funded as a result of solicitations over the years varied from 6 in 1985 to a low of 1 in 1999, with a typical number between 3 and 5.
An indicator of the sustained strength of the cultural impact of an ERC, is whether the ERC characteristics generated by NSF support were strong enough to be sustained by a center once ERC Program support ceased after 10 or 11 years. As the chart illustrates, in 2019 out of the 47 ERCs that have “graduated” from their full term of NSF support, fully 39, or 83%, still existed at that time as self-sustaining centers, most of them with many of their ERC-like features intact. Roughly the same percentage (82%) was found when the study of graduated centers was conducted in 2010.[2] This outcome indicated that the ERC construct was sufficiently viable that ERCs did not generally require NSF “life support” to continue to exist. They could survive on their own with ERC features largely intact in the world. Even one that lost NSF support after a failed third-year renewal review in 2006 was downsized from three partner universities to one—the lead—and was still thriving in 2018 with industrial support and several collaborations with faculty from other universities.
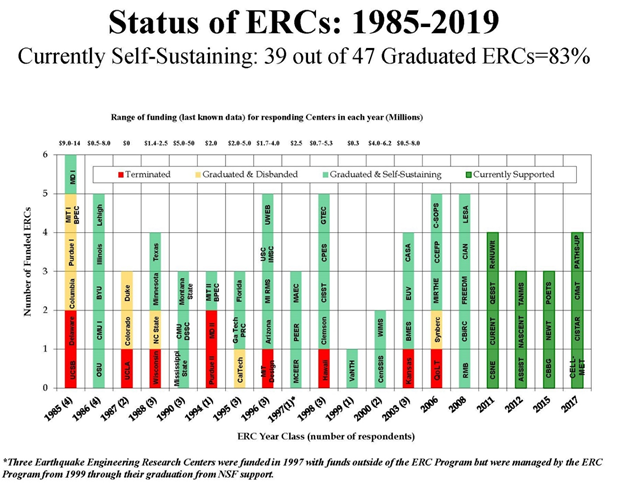
Figure 10-1: Status of ERCs, 1985-2019
ii. Geographic Distribution
In terms of geographic impact in the U.S., ERCs have been established with lead institutions in well over half of the states in the U.S. As Figure 10-2 shows, the states that have not hosted successful ERCs are clustered down the central spine of the nation and across parts of the Rocky Mountain west. The map does not depict states that had only one ERC that was terminated; there are four of these: Delaware. Hawaii, Kansas, and Wisconsin; including those states, 32 of the 50 states have had lead institutions with ERCs. Some states have had more than one ERC: Arizona, California, Colorado, Georgia, Illinois, Massachusetts, Michigan, New Jersey, North Carolina, Pennsylvania, Texas, and Washington.
In addition to the lead university, every ERC since 1996 has had at least one “core partner” institution; many did before then, and most since that time have had three or more. When all the partner institutions are counted, the map of states is considerably more filled in, including the Commonwealth of Puerto Rico. For example, Figure 10-3 shows the map including core partners for only those ERCs current in 2018 (Classes of 2008 through 2017). For this grouping alone, the additional states of New Mexico, Missouri, Arkansas, Wisconsin, Alabama, Delaware, Connecticut, and the District of Columbia are filled in. It is clear that the direct impact of ERCs on universities has been nearly nationwide in scope.
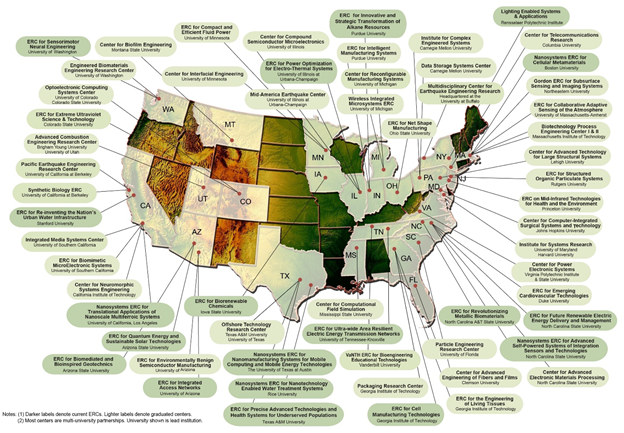
Figure 10-2: All Engineering Research Centers, 1985–2018
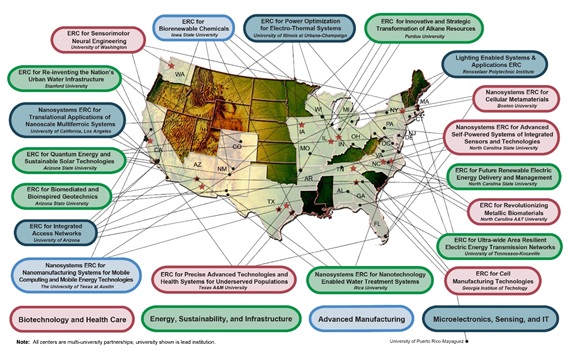
Figure 10-3: FY 2018 ERCs–Lead Institutions and Core Partners
10-A(b) Total Investment by All Partners
The impacts of ERCs are financially enabled by a broad base of financial support from a number of sources, both public and private. (See Figure 10-4.) The great majority of cash support comes from the ERC Program and from other areas of NSF and other government agencies. Industry and the host universities account for most of the remainder, roughly equally in most years. In FY 2018, cash and in-kind support to ERCs from industry totaled $10,223,777.[3]
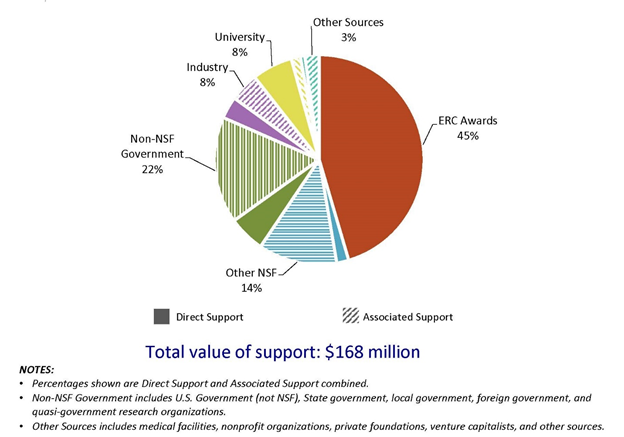
Figure 10-4: Total new cash support to 19 ERCs (FY 2018) (Credit: ICF)
Over the period from 2002 to 2018, a span of years when data were collected and analyzed in a consistent way by the same company,[4] total cash support to all ERCs (which varied in number over time) tended to fall in the range of $130M–$170M, with a maximum approaching $171M in 2013, when there were 20 ERCs. (See Figure 10-5.) Given that the ERC Program support that year was $71.8M, then the leverage to ERC dollars was 2.4 to 1, a figure that generally remains consistent from year to year.
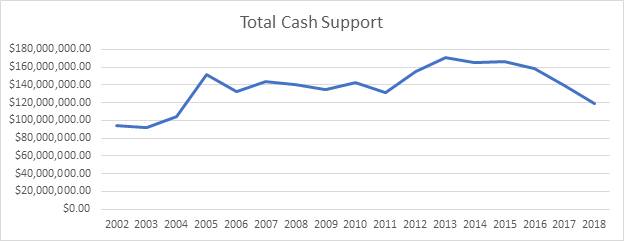
Figure 10-5: Total cash support to all ERCs, all sources, FY 2002 to FY 2018. (Credit: ICF)
10-A(c) Major Impacts/Outputs: Data
Data on many aspects of the ERC Program are collected annually by ICF,[5] a contractor to NSF. They are published on the ERC Association website at http://erc-assoc.org/about/erc_data/program-data. There, aggregate data from the most recent year or across several years between the ERC program’s inception in 1985 and the most recent year are presented in charts and graphs. Using the presented list, a user may go to a specific page, browse through the data page-by-page, or download a pdf of the entire current report.
Some selected highlights for the period from FY 1985 through FY 2018 are given below:
- 851 patents were awarded and 223 spinoff companies were formed.
- 54 new full-degree programs and 31 new minors based on ERC research were established.
- A total of 13,614 degrees at all levels had been granted to ERC students.
- 59% of graduates entered industry, with only 3% of those going to foreign firms.
- The percentage of ERC research and education participants who are women (39% in FY 2018) has increased steadily over the period 1985-2018 and is well above national engineering averages, which are generally under 20%, and includes 47% of ERC undergraduates.
- The percentage of ERC research and education participants who are Hispanics and Latinos (14% in 2018) has been consistently above national engineering averages (about 10% in 2018) over the period 1985–2018 and includes 18% of undergraduates.
- The percentage of ERC research and education participants who are from underrepresented racial minorities (12% in FY 2018) has been consistently above national engineering averages of 3% to 6% over the period 1985–2018 and includes 18% of undergraduates.
- While 10% of undergraduate students conducting ERC research were foreign in FY 2018, 55% of doctoral students were foreign, which was approximately equal to the engineering-wide percentage
- In FY 2018, there were 596 industrial members and financial supporters of 19 ERCs, a number which has been roughly steady since FY 2013 but is sharply up from the several preceding years (encompassing the period of the “Great Recession”).
- Small-company and large-company members of ERCs were about equally represented in FY 2018, at 46% and 47%, respectively (the remaining 8% being medium-sized companies).
- In FY 2018 there were 607 project researchers in ERCs; the most numerous group was Electrical, Electronics, and Communications engineers, at 25%; the next most prevalent group was Bioengineering and Biomedical Engineering, at 16%.
- In FY 2018, the country with the most companies as members of ERCs was China, with 32. Germany was second with 20, followed by the U.K. with 15.
It is evident from the data that the ERC Program has been a strong generator in many areas of academic engineering, including transfer of university-based technologies to industry—and collaboration with industry in general, including small start-ups—the integration of interdisciplinary engineered systems research into the curriculum, and improving access to engineering education for a broadly diverse group of students.
10-B Culture Change in Engineering Stimulated by the ERC Program
10-B(a) Impact on University Culture
As the original NAE guidance made clear, the mission of the ERC Program was essentially to become a “forcing function” for change in the culture of academic engineering in the U.S. Throughout the early years of implementing the mission, it was clear that this change would be challenging but possible. The overviews and analyses of achievements in the preceding chapters on research, education, and industrial collaboration certainly make it evident that the ERC Program has had a significant impact on the culture of U.S. academic engineering and its interface with industry, as initially envisioned. To better understand the degree to which those impacts had been achieved and what more needed to be done, Preston commissioned two important studies, one in the late 1990s and the other in 2003. The purpose of these studies was to gain an independent assessment of how, and to what extent, ERCs achieved the following on the campuses where they were located: (1) impacted the disciplinary culture of engineering and the broader university, (2) developed and sustained a culture of strategic research planning and a focus on engineered systems while retaining a focus on fundamentals, (3) enhanced the interactions with industry, and (4) changed the culture of engineering education to infuse it with a knowledge of systems and industrial practice.
These studies and their major goals were:
- Ailes/Feller/Coward—The Impact of
Engineering Research Centers on Institutional
and Cultural Change in Participating Universities. The goal was to determine the degree to
which ERCs by 1998 had produced or contributed to changes in the institutional
and cultural
norms of academic engineering research, education, and technology transfer
in their
host universities by:
- identifying changes that affected faculty, students, and academic units directly involved in the ERC; and
- identifying changes in the larger university setting, i.e., in the larger set of policies, practices, and behaviors of other parts of the university—i.e., the “spillover” or “externality” effects of the ERCs.[6]
- Currall/Stuart/Perry/Hunter—Engineering Innovation, Strategic Planning in National Science Foundation-Funded Engineering Research Centers. The goal was to study the use of the ERC Program’s three-plane strategic planning framework for developing an ERC’s strategic research plan and its impacts on research publication productivity and technology commercialization (i.e., research application).[7]
These studies and the lessons learned from final reports of some of the ERCs will be used to illustrate the cultural impacts that ERCs made on their home and partner institutions and industry. These impacts were derived from the ERC Program’s requirements that they function with an engineered systems vision, enabled through strategic research planning, cross-disciplinary team research, and partnerships with industry designed to advance all levels of technology and better prepare a diverse engineering workforce for work in industry.
The first generation of 21 “Gen-1” ERCs, established between 1985 and 1990, were pioneers in implementing the ERC construct in an academic engineering culture that was largely driven by the interests of discipline-based departments, where the pursuit of fundamental research in engineering was paramount and the outputs and impacts were measured largely in terms of publications. (See Chapter 3, “ERC Generations.”) Close interaction with industry was generally not fostered by NSF programs, with the exception of the NSF Industry/University Cooperative Research Centers (I/UCRCs) program which began in the 1970s and continues today. The I/UCRCs were focused on problem solving and generally applied research to address industry needs.
To put the ERC Program’s initial charge and operation in context during the 1980s, industry R&D labs supported applied research projects in academic engineering departments; and there were other major efforts by industry to support academic engineering research. These included the Semiconductor Research Corporation (SRC), which was established in 1982 by the Semiconductor Research Association through a Board led by Erich Bloch, from IBM. In 1984, Bloch served on the NAE committee that recommended the formation of the ERC Program at NSF, and in 1985 became the NSF Director. The SRC supported university/industry partnerships in microelectronics-focused research centers through SEMATECH starting in 1985; early ones were located at the University of California-Berkeley, Carnegie Mellon University, and Cornell University. At the same time, Bell Labs and other major corporate research labs were supporting significant programs of academic research. Starting in the late 1970s and early 1980s, the Whittaker Foundation began a program to fund interdisciplinary biomedical research.
NSF’s and industry’s investments in the Gen-1 ERCs established platforms of systems-driven, cross-disciplinary research and education in partnership with industry. , Along with investments made by industry and other Federal agencies, they helped stimulate and facilitate the development of new fields like bioengineering, intelligent manufacturing systems, information systems, and microelectronic processing systems.
With the help of the ERC Program’s Annual Meetings, designed to build an ERC community of shared successes and failures, as well as the post-award oversight teams and NSF ERC Program staff, these centers, their host university administrators, industry partners, and NSF were all learning together how to stimulate and reward a change in the culture of engineering from a bottom-up, single-investigator-driven fundamental research culture to a culture that could sustain partnerships across the disciplines and with industry to span the spectrum from fundamentals through engineered systems and more effectively stimulate technological innovation.
The Ailes study focused on 17 ERCs—a subset of these 21 Gen-1 centers, due to the failure of three of them to pass through their third-year reviews and the termination of one due to malfeasance. Site visits were carried out at 10 of these. The interviews were carried out with the faculty involved in the ERCs and with administrators, including deans, provosts, and vice presidents of research. By the time of the interviews in 1998, one of the Class of 1985, Columbia, was just one year out from its last year of NSF support and the remaining members of that Class were operating with extensions of NSF support post-recompetition: MIT for another 11 years, Purdue for up to five years, and Maryland for three. The youngest set, the Class of 1990, was operating in its eighth year.
In the Ailes study, the “…analysis showed that there (were) few, if any, structural characteristics at the institutional level itself that account for high or low impacts on the culture of the institutions more broadly. The results were widely dispersed over the various institutional types—public/private, small to extremely large, etc. The pattern was also dispersed with respect to variables associated with the ERCs themselves, but a few characteristics here seemed to be at least somewhat correlated with the degree of positive impacts. These included high prominence of the Centers’ educational programs, a high degree of undergraduate involvement, a central campus location, and a high degree of administration interest in and interaction with the Center. With the small number of observations available, however, it is the dispersed pattern over almost all variables that is most striking.”[8]
However, digging deeper into the case studies in the Ailes study, along with Preston’s memory and input from some of the former center directors, it becomes apparent that there were significant academic and industrial cultural impacts across these centers; but those impacts varied across the following features: interdisciplinarity, systems focus, strategic planning, industrial collaboration, and education.
i. ERC Impacts on Disciplinary Culture of Universities
The Ailes 2001 study points to changes in the academic culture resulting from the interdisciplinary construct of an ERC:
The interdisciplinary and collaborative nature of ERC research and education runs counter to traditional notions of individual faculty research results published in a well-defined set of disciplinary-based journals. Hosting an ERC thus required a university to consider and often to adjust its norms and policies for promotion and tenure. Deans, department heads, and ERC directors often reported having to “educate” P&T (Promotion & Tenure) committees about the emergence of collaborative research and publication. Usually a balance was struck, often informally, between a threshold of departmental work and a commitment to ERC research, although some universities did formal restatements of P&T requirements. ERC Directors are often influential in the P&T process, and while a few junior faculty were reported to have been discouraged from participating, few ERC participants failed to attain tenure. In many cases, the ERC participation was perceived as an advantage.”[9]
See Chapter 4, Sections 4-B and 4-C for commentary on the P&T impacts of ERCs.
Following that statement there were important findings about the merging of disciplinary expertise in various ERCs and its longer-term impacts on the host universities. One of the strongest interdisciplinary institutional impacts occurred at the Class of 1985 Biotechnology Process Engineering Center (BPEC) at MIT. There, the Ailes team observed that:
Although MIT as an institution reflected many ERC-like characteristics prior to the establishment of BPEC, the Center nevertheless had significant impacts on the University. These impacts were not confined to immediately participating departments and students, but were reflected in changes of both an institutional and organizational nature within the School of Engineering, in particular, but also within the School of Science. The most immediate impact was in terms of the new collaborations forged between faculty from the departments of chemical engineering in the Engineering School and biology in the Science School. While interdisciplinary research was held to be quite common at MIT prior to the ERC, cross-college efforts were relatively rare.
The model of linkages between biology and chemical engineering developed in BPEC created a broader consensus on the campus of the utility of increasing the understanding of fundamental biological research and methods within engineering more broadly. In 1993, the University began to require that all undergraduates, including those in engineering, take coursework in biology. By 1998 at the time of the SRI site visits, the University was in the process of setting up a new organizational structure within the School of Engineering to formalize the role of biology within the entire engineering curriculum, an effort viewed as largely emanating from lessons learned from BPEC and the desire to embed these lessons in the educational structure of the School of Engineering writ large. Biology had come to be seen as the fundamental core underpinning a great deal of the interdisciplinary problem solving that would be required for engineering advances of the future.[10]
These new collaborations in BPEC, which involved team teaching and team research, helped spawn a new discipline—biological engineering—at the interface of molecular biology and engineering. The groundwork at MIT had been laid by a collaboration between Daniel I.C. Wang, the Director of the original BPEC who had been working at the interface of biology and engineering, and Harvey Lodish, an eminent biologist from MIT’s Department of Biology.
The following is a sample of the significant interdisciplinary impacts of the remaining Gen-1 ERCs, as derived from the Ailes study:
ERC for Intelligent Manufacturing Systems (IMS), Purdue University – Class of 1985
Prior to the establishment of IMS, the Purdue School of Engineering functioned with large disciplinary departments where faculty worked independently in discreet subfields of engineering. As phrased by one academic official, “the ERC proved an ‘existence theorem,’ namely that cross-faculty collaboration could be fostered, succeed, and generate desirable results.” The ERC affected relationships in and across these large departments. For example, faculty in electrical and computer engineering were no longer limiting their interests to robotics, applied controls, or communications but, due to the ERC, were described as working on common problems of manufacturing in ways they would not have without the existence of the ERC. One indicator of increased interdisciplinary interaction, according to interviewees, is that faculty now described themselves in departmental materials and websites as working and teaching in multiple areas and disciplines.[11]
Advanced Combustion ERC (ACERC), Brigham Young University (BYU) and the University of Utah – Class of 1986
The Ailes team observed that the start-up culture at BYU was collaborative and “ground-up,” probably based in the commonality of its shared religious values. Despite this collaborative culture, prior to ACERC the only significant interdisciplinary interaction took place between the mechanical engineering and chemical engineering departments through a combined laboratory. ACERC expanded these collaborations to include seven or eight departments, with the degree of interdisciplinarity determined by the problem at hand. ACERC is said to have produced no paradigm shift, but rather to have been an important catalyst to foster faculty interaction and broaden it to more explicitly interdisciplinary projects. It altered few policies, but changed “practice” in the institution. ACERC has made the University more open to such centers: at least six were under consideration in 1998.[12]
Data Storage Systems Center (DSSC), Carnegie Mellon University (CMU) – Class of 1990
The Ailes interview team noted that at CMU a long-standing “emphasis on interdisciplinarity and industry collaboration is widely held by CMU faculty and administrators to permeate the University culture. Cross-departmental research and industrial support are prevalent in the Colleges of Science and Engineering, due in large part to the University’s strategic plan, which has embraced these modes of research for close to twenty years. Within this culture, the DSSC is seen as a leader in industrial collaboration and interdisciplinary research. Although Carnegie Mellon had a strong atmosphere of cross-departmental collaboration and industry interaction well before the DSSC, the University administration views the ERC objectives as validating CMU’s research culture. DSSC both enhanced the magnitude of interdisciplinary activities among the academic units involved in its programs and reinforced this orientation across the University. Its extensive interactions with industry, including a new ability to integrate longer-term fundamental research with shorter-term applied problems of immediate interest to industrial sponsors, also introduced new possibilities for other academic units in their relationships with industrial sponsors. Faculty report being excited about their involvement with the Center because it gives them fresh concepts to think about that they would never have seen if isolated within their home departments. The prominence of the Center is said also to be a major force in strengthening the College of Engineering’s ranking in U.S. News.”
Given the knowledge gained through this study and the interactions between the Gen-1 ERCs and Gen-2 ERCs[13] at the ERC Annual Meetings regarding how to organize interdisciplinary research to address an ERC’s systems goals, the ERC Solicitations and the review processes were better informed regarding the potential and payoff from interdisciplinary research. The Gen-2 ERCs working at the interface of biology, medicine, and engineering further expanded the field of bioengineering, the role of engineering in information technology, and the interface of earthquake mitigation technology and National Weather Service and local government emergency management decision processes.
In 2004, Preston asked a group of ERC Directors from Gen-2 ERCs and two researchers who focused on interdisciplinary research[14] to provide input to her on the opportunities and challenges inherent in interdisciplinary research, in preparation for an invited plenary address on interdisciplinary research in ERCs at the ASEE’s 2004 convention.[15] Their input pointed to the opportunities shown in Figure 10-6:
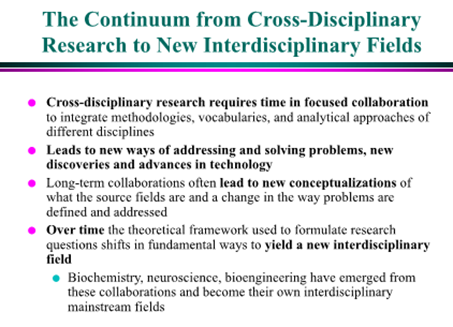
Figure 10-6: Opportunities afforded by an ERC cross-disciplinary structure[16]
Even though all ERCs achieved breakthroughs by working across fields and some of their work laid a foundation for new fields, there were still many barriers in the way as new ERCs were established in universities that had not received prior ERC awards or in universities where the single-investigator culture still predominated. The challenges shown in Figure 10-7 represent an amalgam of Preston’s insights in promoting and funding interdisciplinary research and the ERC Directors experiences in the “trenches” managing ERC interdisciplinary research programs at that time.
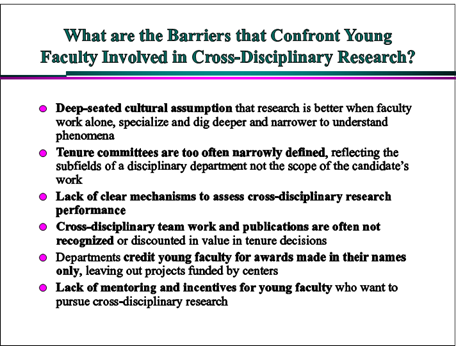
Figure 10-7: Risks for young faculty in ERC research[17]
Figure 10-8 points to the recommendations made to university administrators to smooth the way for faculty and students who saw opportunities at the interface of disciplines and wanted to pursue them and be recognized and rewarded for their efforts by the university systems.
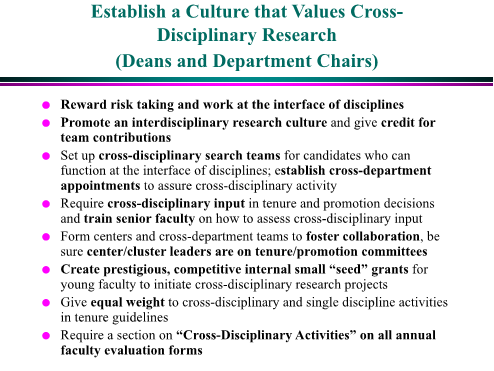
Figure 10-8: Recommendations toward establishing a supportive ERC culture[18]
The Gen-1 ERCs were pioneers in laying the groundwork for teams of faculty to work together across disciplines, motivated by shared goals and a shared vision. They were part of a trend in that period for closer involvement between faculty and industry and they pioneered a new model for engineering graduates: innovators familiar with industrial practice and ready to be productive as soon as they entered industrial positions. The Gen-2 ERCs legitimized strategic research planning from the engineered systems level and implemented complex systems testbeds.
Both of these generations of ERCs worked closely with their university administrators to infuse the academic engineering culture with models of how interdisciplinary teaming could be productive for faculty and users in industry and medicine. By the end of two decades of ERC funding, most ERCs had achieved very strong cross-disciplinary cultures and some had even laid the groundwork for interdisciplinary fields, such as bioengineering and regenerative medicine, or had built pathways for collaboration between engineers and social scientists to improve the utility of technology. In ERCs the systems focus and system-level testbeds were the forcing function to bring the disciplines together and lift the faculty’s sights higher than the project level.
The Gen-2 Collaborative Adaptive Sensing of the Atmosphere (CASA) ERC, Class of 2003, is an example of how the second generation of ERCs could effectively achieve complex engineered systems goals in collaboration with industry and emergency managers. Building and optimizing CASA’s complex systems-level testbed of collaborative adaptive radars required the interface of electrical engineering and atmosphere science skillsets, which resulted in a new cross-disciplinary culture at CASA and its partner universities. In addition, CASA’s systems goals engendered a need for input from social scientists and emergency managers in order to provide operational natural hazards warnings to the National Weather Service and emergency management end users in the most immediately usable fashion. The lessons learned that David McLaughlin, CASA’s then-Director, provided in the ERC’s Final Report in 2013 included the following insights about the role of the CASA system-level collaborative sensing testbed.
The definition of the user relationship was a critical element in the success of the project, since the overarching vision of the center is to create a system that is responsive to user needs. By intensively working on this, CASA was able to shift its focus away from relying only on the research community as users and point the center toward operational users – forecaster and emergency management officials who deliver warning and sound alerts. This, in turn, motivated undertaking additional research to better understand the decision-making of such users during hazardous weather events. The system goal of fielding a real system with real users had the effect of focusing everyone—NSF, the CASA center, the IAB, and peer review groups, etc.—on the ‘end-to-end’ aspects of the problem, rather than limiting the focus to the radar, weather, or other single-disciplinary aspects of the problem. The result is a deployed system that is currently demonstrating capabilities fundamentally beyond the present state of the art, in which the ultimate end users are intimately involved in the research and system design. This project demonstrated that CASA’s concept works and that the team could execute innovative, complicated projects of social and scientific value.[19]
Building and sustaining the type of interdisciplinary culture that CASA represented took leadership at many levels and the ability of faculty to bridge beyond their disciplinary comfort zones, bringing their skills and perspectives to bear on problems that would be intractable without collaboration across disciplinary boundaries. ERC funding motivates this collaboration and sustains it over 10 years, but it cannot thrive over the long run unless the departments and schools are willing to reward and promote this collaboration.
To provide an update on progress and challenges in building cross-disciplinary cultures in Gen-2 ERCs, in 2012 Preston asked the bioengineering/biomedical engineering ERC Center Directors to reflect on the issues involved in joining engineering and biology to achieve ERC technology goals. At that time, a team led by Dr. Mark Humayun, MD, who is a Professor of Medicine and Engineering at the University of Southern California and the Director of the ERC for Biomimetic Microelectronic Systems (BMES), had developed theArgus II retinal implant, which was in the FDA approval process for clinical use in the U.S. Argus II is a prosthesis to bring a degree of restored sight to patients with retinitis pigmentosa and other diseases that deteriorate or destroy the retina. It was approved in 2013.[20] This achievement was more than 20 years in the making. The vision could not have been achieved without research at the intersection of biology, medicine, and engineering because Argus II required a system of devices that could transmit images to an image processing chip that would then transmit those signals to the optic nerve, thereby replacing some of the lost functionality of the retina.
The achievements of BMES and several other ERCs working at the interface of biology and engineering, or for that matter all the other ERCs, required building a culture of integrative collaboration—a new research ecosystem—where:
- Scientists come to understand that it’s OK if their discovery and exploratory modes of research can be directed to the solution of important problems;
- Engineers learn enough about the sciences and medicine to draw on that knowledge to achieve targeted technology outcomes;
- The team learns to challenge each other when new barriers arise;
- Students bring disciplinary depth and gain an enriched educational/research environment at the interface of disciplines;
- The team has contiguous space, places for faculty and students to socialize outside their disciplinary spaces;
- The team has strategic targets, periodic reviews of delivery, course correction changes, etc.;
- Collaborative projects must give each researcher opportunities that can still be valued within their disciplinary research spheres, to be sustained in the long-run;
- Creation of new journals and new subsections of major journals will stimulate the further development of new fields emerging from the research.
To verify these assumptions, Preston challenged a group of ERC Directors whose centers worked at the interface of biology and engineering to address the differences between how biologists view their research (discovery) versus engineers (control and/or deliver functionality) and how to join them to address technology goals. Their responses centered around ensuring clear and frequent communication, ensuring that conflicting participant needs are addressed and met, having a big and challenging goal that participants have the freedom to approach as they see fit, and providing contiguous space if at all possible. The full responses are in the file “Biologists x Engineers.doc.”
These findings pointed to a significant base of knowledge among ERCs about how to structure cross-disciplinary collaborations to address the systems goals, thereby creating a new space for the growth of interdisciplinary collaboration.
In 2018, Preston asked 18 directors of the more mature Gen-2 ERCs and early classes of Gen-3 ERCs[21] to reflect on the outcome of her 2004 survey of ERC Directors on the interdisciplinary culture of their centers and their universities (described earlier in this section) and to provide insights and recommendations based on their more recent experience. These directors were from ERCs in the Classes of 1990 through 2012. Surprisingly, only three responded. These were: Russ Taylor, Director of the Computer Integrated Surgical Systems Technology ERC (CISST), Class of 1998 (Gen-2), Johns Hopkins University (JHU); Brent Shanks, Director of ERC for Biorenewable Chemicals (CBIRC), Class of 2008 (Gen-3), Iowa State University; and Greg Carman, Director of the Nanosystems ERC for Translational Applications of Nanoscale Multiferroic Systems (TANMS), Class of 2012 (Gen-3), University of California, Los Angeles (UCLA).
These directors agreed with the earlier classes of ERC directors that cross-disciplinary research:
- requires time in focused collaboration to integrate methodologies, vocabularies, and analytical approaches of different disciplines;
- leads to new ways of addressing and solving problems, new discoveries, and advances in technology;
- leads, in the long-term, to new conceptualizations of what the source fields are and a change in the way problems are defined and addressed; and
- shifts, over time, the theoretical framework used to formulate research questions in fundamental ways that can yield new interdisciplinary fields.
Russ Taylor added that “a mixture of translational and ‘fundamental’ research, such as is fostered by ERCs, is often highly productive for both. The translational aspects—i.e., the need to produce something that is useful in the real world—often helps one focus on the need to develop new fundamental knowledge—that is, to identify a knowledge gap that must be closed in order to resolve an implementation gap.” He added that this need can drive the search for new collaborators to fill that gap, often broadening the impact of an ERC. An ERC that is focused on an emerging technology, such as robotics, can also stimulate further cross-disciplinary research. He believes that the cultural barriers against cross-disciplinary research have come down as universities better understand that working in teams can be productive, a change that has often been driven by new faculty from industry, where the culture of bringing people together to solve problems or work on opportunities is more prevalent. In addition, barriers to tenure and promotion have come down as well—at least at JHU, “where ad hoc committees have a very strong role in promotion cases and the Dean selects people from multiple departments to span the candidate’s research focus. Again, I am sure this varies greatly from school to school and university to university.” In addition, the culture established by the CISST set up mentoring opportunities for younger faculty, which have now spread across the School of Engineering.
Greg Carman added the following insights about cross-disciplinary research in the UCLA culture: “Basically, in my community we have accepted that cross-disciplinary research is important and we have to be vigilant so our university organizations do NOT erect barriers preventing this type of collaboration. My community has accepted that research problems our society is facing are difficult and that group research represents a better mechanism to solve complex problems; accordingly, reward systems are in place. While management has accepted this as a fact, the individual faculty members (sporadically) still put up resistance. I personally attribute this to the fact that some Profs still train their PhDs with an “individual” focus rather than a “group” focus. So I think the ERCs have helped change the management culture, but this change has not trickled down to all engineering faculty in the universities.”
This input, while small in numbers, reflects the changes in university culture that have enabled interdisciplinary research to flourish alongside disciplinary research in a broader and more inclusive academic research culture.
ii. Evolution of Engineered Systems Thinking Across the ERC Generations
ERCs are required to function with systems-level goals driving the formulation of research programs and proof-of-concept technology testbeds that have had a significant impact on center researchers and their industrial partners. However, the Ailes study of the Gen-1 ERCs found that most ERCs’ systems research has had little on-campus cultural impact outside their ERC circle of participants. The Ailes study findings point to the following lessons:
The generic objective of achieving system-level goals was shared widely among participants at every host institution, but interviews seemed to indicate that the terms ‘engineered systems’ and ‘systems approach’ meant all things to all people. Consequently, the specific definition and operationalization of the concept varied both within and across host institutions. A few of the Centers, such as Carnegie Mellon’s DSSC and the Mississippi (State) ERC, had an engineered systems orientation viewed internally as the core feature of the ERC that had led to impacts in other areas. More frequently, Centers reported difficulty in formulating what constituted an appropriate engineered system that met NSF agreement. This was particularly true of Centers that have more conceptually based research agendas, such as Maryland’s Systems Research ERC. Regardless of the degree to which the engineered systems approach was embraced within the ERC, it had negligible effects on other activities, even within the College of Engineering.[22]
The following are examples of their findings for Gen-1 ERCs.
At the Brigham Young University/University of Utah Advanced Combustion Engineering Research Center (Class of 1987), the “objective was to provide industry with comprehensive models of large-scale combustion systems based on an experimental approach in which models of smaller components would come to be integrated into a larger ‘system.’ Center respondents reported that a ‘paradigm shift’ in industry toward using the more comprehensive models as tools did occur, and took credit for having introduced this perspective.”[23]
The systems approach at the University of Colorado, Boulder/Colorado State University Optical Computing Systems (OCS) ERC (Class of 1987) resulted in a fertile cross-disciplinary culture where one researcher note to the Ailes/Feller interview team that “materials researchers keep device researchers realistic in what can be built, while device researchers provide feedback to materials researchers on the effectiveness of their materials and the importance of different parameters. Similar interaction occurs between systems designers and device researchers.” Faculty participating in OCS described several similar impacts upon research. They reported becoming accustomed to working in different ways, to working with other departments, and to working with industry. One faculty member noted that after he had become part of the OCS, his team’s work became very systems oriented and his research began to take into account the constraints of other disciplines. For example, in developing packaging material for a product, his team understood that the material could not adversely affect the properties of the optics. The interaction was said to have helped define interesting research projects. Another example cited was the broadening of one ECE (Electrical and Computer Engineering) researcher’s approach: “…his involvement through OCS with a faculty member in chemistry led him to explore the use of organic photoconductors to drive photoelectronic devices, a technique used by chemists. He had originally been taking a different tack but once introduced to the technique, ended up hiring a chemistry postdoc to assist in his research. He observed how he and the postdoc had battled over terms, but in the end their collaboration had led to the redefinition of the technical problem.”[24] Preston notes that these findings point to a more pervasive impact at CU because of the interdisciplinary, systems approach of OCS, which also extended to its partner institution, Colorado State University (CSU). In fact, two faculty at CSU, Jorge Rocca and Carmen Menoni, who were junior faculty in the late 1980s in OCS, later teamed up with members of the CU physics department to submit a proposal for a new ERC, the Extreme Ultraviolet Science and Technology (EUV) ERC, which was awarded in 2003.
The Ailes interviews also found that “From the perspective of several interviewees, the systems focus of the DSSC (the Data Storage Systems Center), established in 1990 at Carnegie Mellon University, contributed to its having a larger impact on CMU than did the earlier MTC (precursor to DSSC, the industry-funded Magnetics Technology Center). It allowed the Center to change its research from incremental improvements to one of reinventing an entire technological system. This orientation, in turn, meant leapfrogging incremental improvements in separate components. It was thought that companies could not attempt this type of research. The systems approach thus permitted the DSSC to combine long-term fundamental research with specific R&D applications of high saliency for industrial sponsors. The DSSC is now being approached by industry for more typical short-term industrial research projects in this area, which is attributed largely to its earlier research accomplishments at a more fundamental level. The spread of a systems orientation outside of DSSC to other academic units, however, is less evident, as might be expected.”[25]
The strong role of systems in these three Gen-1 technology-focused ERCs is in contrast to the Class of 1985 Biotechnology Process Engineering Center at MIT, which was focused at the interface of biology and chemical engineering to advance mammalian cell processing technology. A reactor is essentially a mini-system, but the ERC was often confused about its systems focus. The Ailes study found that “In its broadest usage, BPEC was held to represent a systems orientation because its research projects went from biological properties to pharmaceuticals to delivery. However, in general, given the relatively strong ‘science-orientation’ of BPEC compared with most ERCs, BPEC was thought to be less systems oriented than others.”[26]In contrast to this observation, upon reflecting on her experience with BPEC and a description of its processing system in Steven Currall’s book, Preston views the systems challenge at BPEC as one of turning mammalian cells into mini-factories, directing cultured cells to “produce desired proteins in reactors (mini-processing systems) that functioned to prevent in-vitro cells from sticking to bubbles formed in the processing and exploding upon reaching the surface.”[27]
The Ailes/Feller study found that systems was also a point of contention between NSF and the Class of 1985 Systems Research Center (SRC) (after 1988 called the Institute for Systems Research [ISR]), at the University of Maryland. “However, coming up with a consistent, agreed-upon definition of what constituted systems engineering was a recurrent and thorny issue between the Center and NSF. The ‘systems perspective’ which ISR participants associated with NSF’s intent for the ERC Program seemed to combine a vertical integration that ran from basic science to the marketing of an application with a horizontal perspective that involved the cross-disciplinary integration of relevant research domains. NSF was seen as defining systems in terms of applications of engineering research to specific topics, whereas ISR wanted to highlight the methodological research that was at the core of their program.”[28] Preston points out that one of the reasons the ISR was funded over a competing systems proposal was that the ISR proposal proposed development of systems engineering grounded in applications rather than remaining at a theoretical level, which was perceived to be the motivation of the competing proposal. Assuring that the SRC/ISR grounded their research in driving systems applications was a motivating factor in the NSF oversight as well.
As knowledge of systems progressed, by and large the Gen-2 ERCs (Classes of 1994–2006) functioned with a better-defined set of systems goals than the Gen-1 ERCs. Preston believes this was due to the ERC Program’s improved definition of systems as engineered systems, and to the improved definitions of testbeds that were introduced in the Gen-2 period and included as requirements in the ERC Program Announcements of that period. Examples of those definitions follow: “An engineered system is derived from integrating a number of components, processes and devices to perform a function. The system may be living or inanimate in origin. It must be complex and challenging enough to justify a ten-year program of research. Analysis, modeling, or development of the individual components of a system, without their integration into a complex engineered system, is not an appropriate focus for an ERC.”[29] And “Proof-of-concept testbeds in ERCs are used to explore an ERC’s next-generation engineered system to determine if all components work together as planned and the system is feasible. These testbeds help to ensure that the research outcomes are integrated and tested and supply a framework for faculty, students, and industry representatives to work together and gain a better understanding of the realities of the system they are exploring and demonstrating.”[30] Finally, the introduction of the ERC 3-Plane Strategic Research Planning tool in 1998 proved to be a more effective approach for ERCs to use to structure their research programs to address their engineered systems goals.
The following are examples of the power of the systems construct when effectively executed through an ERC.
The distributive collaborative adaptive sensing networks designed and tested by the Collaborative Adaptive Sensing Systems (CASA) ERC to observe, predict, and respond to atmospheric hazards are an example of one of the most complex and yet effective engineered systems developed by an ERC. The CASA system encompassed radars as well as associated computation and communication infrastructure that turn observational data into actionable information. The ERC’s Director, David McLaughlin, remarked in the ERC’s final report that “Designing, developing, implementing and operating a complex engineered system as CASA’s IP1 test bed demonstrates to the academic community the necessity of Systems Engineering leadership and design process and a means to do that within an academic setting, where curiosity, academic freedom, risk-taking, and students’ theses all need to be supported.” “The host academic Department for CASA is the Department of Electrical and Computer Engineering at UMass Amherst. Asked to comment about CASA’s impacts on departmental culture, Department Head Dr. Chris Hollot wrote: ‘Over its lifespan, CASA provided a drumbeat that impelled ECE to: think bigger, think systems, think interdisciplinary. We have no doubt that ECE’s recent and burgeoning interdisciplinary research initiatives in nanotechnologies, security and privacy, and personal health monitoring all owe their broader perspectives to the CASA model. CASA positively impacted ECE’s tenure track faculty—to think (and act) bigger, broadly and across boundaries.”[31]
The Biomimetic MicroElectronic Systems ERC at the University of Southern California (Class of 2006) produced an excellent example of an engineered system designed to restore some sight to patients impacted by retinitis pigmentosa, shown in Figure 10-9. This Gen-2 ERC also reflects the strengthening of the systems concept in biomedical ERCs and the ability of one Center Director, Professor Mark Humayun, to envision such a technology system and then orchestrate the collaboration of an interdisciplinary team of researchers across engineering and medicine at three universities, technologists in industry, and ophthalmic surgeons around the world. Together they explored the concept, developed prototype systems (dubbed Argus II), experimented with them as proof-of concept testbeds through a spin-off small business (Second Sight, Inc.), and implanted the Argus II system in a cadre of courageous patients. Initially, Preston remembers the patients viewed themselves as research subjects and were satisfied with that role, and were even delighted with their ability to see light and shadows. Over time, as the technology developed, the earlier and then newer subjects actually began to regain more and more sight while continuing to contribute to the continual refinement of the technology. In 2013, FDA approved the implant for clinical use; and in 2016, Humayun received the National Medal of Technology and Innovation from President Barack Obama for his innovative work leading to the development of Argus II.
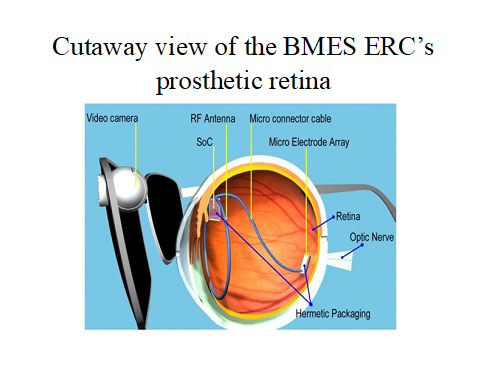
Figure 10-9: The BMES prosthetic retina (Credit: Second Sight)
iii. Role of Testbeds in Changing the Culture of Academic Engineering Research
NSF’s goal for the development of testbeds in ERCs was to impact the culture of academic engineering by providing faculty and students with experience in testing their ideas for technology against the “realities” of implementation on an academic scale. The understanding of testbeds in academic research grew over time in ERCs as engineers began to understand how to work together across disciplines, exploring new technological frontiers, applying first principles in engineering and defining new ones as barriers arose, eventually advancing enabling and systems technology in testbeds. This full exploration of enabling and systems testbeds in ERCs generated another culture change in academic engineering. As Ken Wise noted in his final report to NSF on the ERC for Wireless Integrated MicroSystems (WIMS – Class of 2000):
Academia is oriented strongly toward individual accomplishment, and faculty members accordingly have a strong inclination to work with their graduate students on individual projects. Such projects produce very worthwhile advances in narrowly focused areas, but may not go much beyond that. They do not often rise to the systems level nor do they normally involve real teamwork among a group of faculty members. As a result, (ERC research) thrusts tend to collapse into worthwhile individual (but mostly non-interacting) projects. In contrast, testbeds cut across many projects and thrust areas; they not only are essential in terms of promoting faculty interactions, they force them. They require teamwork in which each project must set aside some of its own work to do the things needed to realize the system. While even with testbeds doing systems is not easy, without them it would be impossible.[32]
In a lecture on his 60-year career in engineering, Wise summed up the challenge of engineering as: “We can solve complex problems if we can put stuff together and make the pieces work together.”[33]
Wise’s leadership of the WIMS ERC was a logical step in his career and in the advancement of wireless integrated microsystems because of his experience in industry in the early years of his career, his continued support from industry over time, and NIH support to advance his team’s work on neural probes. Building on the prior work of the Wise team, enabled by the system perspective of ERCs and its long-term funding model, the ERC developed microsystems advances such as:
- Miniature chromatography-based gas analyzers that can sense the presence of biomarkers for tuberculosis and lung cancer[34] in exhaled breath and are also a gateway for worldwide pollution monitoring. This technology is being driven to ever-higher analysis precision, lower power, and smaller size and is being commercialized by a number of companies.
- A hermetically sealed 24/7 intraocular pressure sensor for the improved treatment of glaucoma that was developed in collaboration with the Kellogg Eye Center at the University of Michigan[35] and is now being commercialized.
- A wireless cardiovascular stent capable of detecting arterial re-stenosis,[36] featured on the cover of Annals of Vascular Surgery (September 2005).
- A hermetically sealed, thin-film, high-density 32-site cochlear electrode array allowing closed-loop insertion and more precise locating of the stimulation peaks along the frequency-position map of the cochlea, enabling deeper placement and broader perceived frequency range.[37] This technology is being commercialized.
- Wireless implantable neural interfaces,[38] including 64-site wireless cortical recording arrays for mapping low-frequency potentials and single-cell spike activity at the neuronal circuit level as well as 256-site active stimulating arrays. This work was an important foundation for the present worldwide activity in neural interfaces for neuroscience and, eventually, neural prostheses.
Wise voiced an important insight regarding systems testbeds that has critical implications for the staffing of universities committed to engineered systems research and technology: “A lot of systems work doesn’t produce papers, at least not directly, and both students and postdocs are temporary employees. The creation of systems requires an infrastructure of staff engineers that can take partially-working prototype devices and build systems out of them. Without such an infrastructure, work in academia at this system level is not possible, and yet normal grants and contracts do not typically support such staff (and faculty rarely even ask for them). ERCs are essential in supporting some of this infrastructure to facilitate work at the system level.”[39] Thus a critical lesson learned from ERCs with robust testbeds is that they cannot be “built” without technical staff. ERC Directors should understand that “soft money” technical staff are necessary to build these testbeds because most graduate students don’t stay long enough to see the testbeds reach proof-of-concept. In addition, in some cases of long-term use of the testbeds for research, funds need to be set aside to maintain them in order to enable fuller exploration.
Testbeds were a critical component of the work of the Center for Structured Organic Particulate Systems (C-SOPS), which focused on advanced pharmaceutical manufacturing systems, including continuous manufacturing systems for solid dose medications, which make up 85 percent of all prescriptions dispensed to humans. Contrary to traditional and inefficient pharmaceutical industry practice that uses empirical, trial-and-error batch processing based on a single chemical entity, C-SOPS uses the understanding of the impact of chemical formulation, microstructure, morphology, and molecular interactions across a broad range of pharmaceutical products to predict a final product’s performance when manufactured in a continuous manufacturing system. These new manufacturing methods are not specific to one pharmaceutical product but are applicable to a wide range of products available in solid dosage forms. Economic benefits include a reduction in time and costs associated with product and formulation development and improved product quality and new personalized products for consumers.
In collaboration with its industrial partners and with augmented funding from an ERC Innovation award in 2009, C-SOPS built a testbed to demonstrate the feasibility of continuous manufacturing to bring continuous tablet manufacturing closer to commercialization. This involved the integration and optimization of models and in-line sensing strategies for optimal process control. In partnership with ERC member companies, exploration and demonstration of the continuous processes became available broadly to the pharmaceutical industry and pharmaceutical researchers worldwide. See the discussion of the C-SOPS continuous manufacturing testbed in Chapter 5, Section 5-D(b). Also see Chapter 11, Section 11-C(b) for a discussion focusing on the industrial applications of continuous manufacturing in the U.S. arising from collaborations with C-SOPS.
According to Fernando Muzzio, the Director of C-SOPS, based on their research and new manufacturing processes the C-SOPS team spearheaded the formation of the International Institute for Advanced Pharmaceutical Manufacturing.[40] The result was the initiation of 10–12 new pharmaceutical manufacturing programs in universities worldwide, including the C-SOPs team at Rutgers, Purdue, and UPRM and universities in the UK, Belgium, Austria, China, and Ireland. This institute is joining the centers together as a liaison institute. They hold joint workshops and companies can now work across the centers. (See https://www.i2apm.org/index.php)
The most significant domestic accomplishment of C-SOPS is its impact on the U.S. FDA. The FDA has been involved with the C-SOPS team from the time of the generation of its proposal for an ERC in 2005 because the leadership of FDA was interested in modernizing pharmaceutical processing. Once underway, the C-SOPS team and their industrial partners remained in close contact with FDA and around 2015, FDA formed an internal group, the Emerging Technology Team, to speed up the evaluation of company applications for approval of emerging manufacturing technologies. They have issued a special review process and guidance on how to engage in emerging manufacturing technologies. Janet Woodcock, who then served as the Director of the Center for Drug Evaluation and Research at FDA, asked the C-SOPS team in 2015 to develop a best practices guide on continuous manufacturing. C-SOPS submitted it to FDA in 2016 and the report is part of the input FDA will provide to the ICH in its 2019 initiative to generate a global guideline for continuous manufacturing, which is expected to be issued in 2020. (The ICH is the International Council for Harmonisation of Technical Requirements for Pharmaceuticals for Human Use.)
C-SOPS efforts in this area were recognized by the White House Advanced Manufacturing Council, which in its 2016 report identified Continuous Pharmaceutical Manufacturing as one of just five “Emerging Technology Priorities” and listed C-SOPS as the leading example of successful federal investment. [41]
Moreover, regulatory agencies in Asia have also launched initiatives in continuous manufacturing. Early in 2018, the Chinese FDA instructed leading pharmaceutical manufacturers to embrace continuous manufacturing. More recently, in December 2018, the PMDA in Japan co-organized a conference with the newly minted Consortium for Continuous Pharmaceutical Manufacturing in Japan Working, where C-SOPS director Fernando Muzzio provided the keynote presentation focusing on practical implementation of continuous manufacturing Impact of ERC Strategic Planning on University Research Cultures.
Strong Partnership Aids in Response to Hurricane Maria The Center for Structured Organic Particulate Systems (C-SOPS), based at Rutgers University, has been a world leader in research and industrial collaboration aimed at improving the effectiveness of pharmaceutical manufacturing, especially in the development of technologies for continuous manufacturing of both tablets and capsules. C-SOPS partnered with the University of Puerto Rico at Mayaguez UPRM) and with Johnson & Johnson (J&J) to support the company’s efforts to implement this new technology in-house. J&J invested $15 million dollars in a commercial continuous manufacturing pilot line, based on a C-SOPS design, in Gurabo, Puerto Rico. When Category 4 Hurricane Maria hit Puerto Rico in September 2017, it devastated the island’s buildings and infrastructure, including the power and communications grids. UPRM’s campus was extensively damaged and the university shut down for a considerable period. The C-SOPS team based at UPRM received high priority in recovery and were provided with generators to keep the research going. C-SOPS industrial member companies reached out to the team, providing funds to bring UPRM students to Rutgers to continue their studies and research. Rutgers provided $1M to the students in the form of tuition waivers. UPRM professor Rudolpho Ramanach and four students spent the summer of 2018 at Rutgers so they could continue working, as his lab was destroyed. This is an example of university-industry partnership at its best, and just one example of the kind of reach and impact that ERCs, working in tandem with industry, have had over the years. |
As detailed in Chapter 3, Section 3-B(c), ERCs have been required to function with strategic research plans since 1987, and since 1998, have been required to use the ERC Program’s three-plane strategic research planning tool. The Ailes study of Gen-1 ERCs focused on ERCs that had never functioned with the three-plane chart, or for only one year, as the interviews were carried out in 1998. The Ailes study pointed out that “A key distinguishing feature of an ERC is strategic planning of the research, education, and technology development and commercialization activities of the Center. The ERCs themselves are required to develop their initial strategic plans in the first 3 months and to submit annual updates of their strategic plans as part of their Annual Report to NSF. In the vast majority of cases, the Centers had learned to value this planning process as a sufficiently important determinant of their future research direction that it remained a key management tool even when it was no longer required once the Center’s ERC Program funding had come to a close. However, even in cases where departments, colleges, and the host university’s central administration engaged in voluntary or required planning exercises, the ERC was not regarded as an influence. This was not surprising because in most cases, higher level planning processes had been instituted prior to the award of the ERC, and the ERC’s process had limited applicability outside of the context of cross-disciplinary engineering center research operations.”[42]
Regarding broader institutional impacts of Gen-1 ERCs’ strategic planning, the Ailes study found that “’Strategic planning’ writ large has become a generic descriptor—a “buzzword”—for the processes underlying institutional change or reorganization in many public and private sector organizations over the past decade or so. It is unlikely that this type of generalized organizational planning bears much resemblance to the ERC Program’s strategic planning objective for a funded Center, which is much more specific and much more tailored to the related Program objective of the Center’s specific engineered system focus driving the entire ERC’s activities. The ERC Program goal that ‘An engineered systems focus and strategic planning drive an ERC’s research’ is directly applicable only in cross-disciplinary engineering center-type research operations similar to the ERCs themselves; so it is therefore not too surprising that few, if any, spillover effects of either of these ERC characteristics were found on the campuses examined in this study.”[43]
The introduction of the three-plane research planning tool for Gen-2 ERCs had a significant impact on the intellectual structure of ERCs because it strengthened a center’s ability to communicate how its engineered systems vision structured and motivated the research goals at the fundamental science and enabling technology levels. This succinct expression of the vision led to stronger faculty and industry partner engagement in strategic planning in most ERCs. However, since Preston was concerned about the degree to which strategic planning was or was not ingrained in ERCs and the effectiveness of the three-plane framework, she commissioned a study to shed some light on those concerns. The Currall 2005 study of the use of the three-plane chart within ERCs found that “most ERCs viewed strategic planning and the three-plane framework as valuable.” By that time, strategic planning also was becoming more acceptable in university and department planning in colleges of engineering nationwide. ERCs used the three-plane framework in their initial proposals to the ERC Program. The leaders of these ERCs were familiar with strategic planning, either through experience in industry, leadership development, involvement with strategic planning at the school or university level, or self-education. Due to these broad perspectives, these leaders found value in top-down planning of the research endeavor. They also became champions of strategic planning and the three-place framework in their conversations with faculty members with the ERC.[44] When there was strong acceptance of planning, the study found a number of themes related to acceptance, as many interviewees commented that the three-plane framework helped them:
- organize the ERC and operations with research thrusts;
- organize the overall mission of the ERC;
- avoid distractions of other types of technologies that could be funded yet, were not core to the mission of the ERC;
- allocate resources; and
- communicate the usefulness of their work, i.e., deliverables.[45]
The study also reported that some leaders found the three-plane framework less valuable, often because they were still learning how to use it at the time of the survey. Currall found that “ERC leaders usually gained more appreciation for this planning tool as they learned more about its purpose and intended use and value.”[46] In one case, a pre-existing plan was retrofitted into the three-plane chart and no real changes in future-oriented thinking occurred. In that case, the framework was used primarily for communications to NSF. Thus, the three-plane chart was decoupled from the organization and functioning of the center, confirming what Preston had observed at a few ERCs. For some ERCs that started out as described above, “when the three-plane chart had a champion who was successful in communicating its value, significant improvements in coupling the plan to the vision and the research program occurred.[47]
Still a third set of ERC leaders—generally from more “science-driven” ERCs—placed more of their emphasis on curiosity-driven research rather than technology or systems-driven research.[48] The study found that these leaders did not involve faculty in deriving the three-plane framework for their ERCs and the intended cultural impact within those ERCs was therefore limited.
Preston funded that 2005 study because she was concerned from reading annual reports and attending site visits that some of the ERCs were approaching the use of the three-plane chart as an exercise in compliance with a “government” requirement. For some that was the case, but for most the three-plane chart proved to be an asset for their ERCs—and in fact, eventually in many it was eagerly adopted and utilized. It is important to point out that some resistance came from faculty who felt that the framework was antithetical to creativity. Preston never intended “to replace natural idea-generation of scientists (and/or engineers) by introducing the three-plane framework. It was intended for coordinated planning yet cannot take the place of the natural creative instincts of scientists and engineers. The creative skill of scientists and/or engineers must be taken as a given and the three-plane framework should work in concert with this.”[49]
A compelling argument for the value of the ERC Program’s research-to-innovation construct through the three-plane strategic planning framework comes from the analysis of innovation and the ERC construct in a book authored by Steven Currall and his colleagues. They credit Preston’s development of the framework as a tool to guide ERC leaders on how to move inventions derived from fundamental research toward proof-of-concept—technical demonstrations providing evidence that abstract ideas can have commercial potential. This is the foundation for their view of ERCs as vehicles for “organized innovation.”[50]
iv. Integration of Research and Education
Starting in 1985, ERC research and education programs have been designed to create a cross-disciplinary, team-based culture for engineering education. At the same time that successive classes of ERCs were stimulating these changes, ABET requirements and strong engagement of companies in changing engineering education through organizations like the ASEE were also impacting engineering education at universities on a broader scale than could be achieved by a single ERC.
The ERC culture is different from the single-investigator culture because an ERC’s next-generation engineered systems research is infused into curricula for students at all levels and for practitioners.
The goal of the ERC Program is to produce a new and diverse generation of leaders who are more effective in industry in a global economy. The ERC educational culture provides undergraduate and graduate students with the opportunity to:
- join a university/industry team guided by a vision and strategic plan;
- participate in research projects that draw knowledge from diverse science, engineering, business, and education disciplines;
- work in cross-disciplinary teams; and
- work on the development of engineered systems and validation in proof-of-concept testbeds.
This experience gives them the capacity to identify and realize opportunities, not just solve problems, and affords them time spent on-site in industry and with industrial mentors, working on joint projects. In addition, to assure a continued flow of capable students into engineering, ERC outreach efforts are designed to infuse engineering knowledge and experience into pre-college education to attract young students to engineering and to advance the skills of the technical workforce.
The Ailes study found that for Gen-1 ERCs, “Education was the area in which the most widely spread impacts of ERCs were discernible on the 16 university campuses covered in this study. Although the effects of ERCs per se were often difficult to unravel from the many concurrent influences pressing for change in science and engineering education during the last decade, particularly at the undergraduate level, in every case but one at least some changes in the direction of increased interdisciplinary exposure, team-based research experience, industry interaction, and/or undergraduate involvement research was at least in part attributed to the models set forth by the new curricula and courses, Research Experiences for Undergraduates (REU) programs, seminars and workshops, and other educational activities initiated by the ERCs.”…“While the ERC efforts came at a time when other forces were pushing engineering education in similar directions, there were a few cases in which the ERC was cited as the only locus for undergraduates to gain exposure to research. Industry was reported to find students from these programs better prepared for jobs in industry, and as having a better sense of what a career in industry was likely to be like, confirming other studies’ findings in this regard.”…” Graduate student involvement in an ERC is unique in several respects. First is the degree of cross-disciplinary interaction and exposure through work on ERC research teams that generally involve faculty and students from disciplines other than their own fields of concentration. A second difference is that ERC students typically have considerably greater interaction with industry than is the norm. ERCs were often credited with serving as a major attraction in the recruitment of high-quality graduate students, especially within those departments most directly impacted.”…“The changes attributable to the ERCs were most clearly evident in those departments with direct participation in the Centers, but generally were also apparent to at least some degree throughout the colleges of engineering. In some cases, the educational impacts of the ERCs were experienced as campus-wide phenomena, literally affecting practically all colleges and departments throughout the university.”[51]
One example of a broader university-wide impact comes from the Institute for Systems Research (Class of 1985 and 1994) at the University of Maryland. In the College of Engineering, “…ISR’s leadership in fostering interdisciplinarity was sufficiently evident that it was described as having been requested to take on leadership for nearly all cross-disciplinary activities on campus.”….“The Dean turned to ISR as the appropriate unit in the University to administer this interdisciplinary program. Other new interdisciplinary programs have been assigned to the Institute, whether or not they have been generated from the Institute’s own research efforts and proposal.”…..”A highly significant educational innovation … was an undergraduate honors program called Project Gemstone. This project was initially supported with industrial funds and a seed grant from the ERC Program. It was partly inspired by the Center’s mode of team effort, as well as by courses developed through the University’s participation in an NSF Engineering Education Coalition that put emphasis on teaming and early design projects in the engineering curriculum. Incoming freshmen are specifically recruited into the program and assigned to teams that work together throughout their undergraduate career. The teams cross all disciplines and colleges—not just engineering. The program has been very successful, attracting increasing numbers of top-ranked freshmen.”[52]
The Gemstone Honors Program continues today and is advertised to students considering attending the university as follows: “The Gemstone Program at the University of Maryland is a unique multidisciplinary four-year research program for selected undergraduate honors students of all majors. Under guidance of faculty mentors and Gemstone staff, teams of students design, direct and conduct significant research, often but not exclusively exploring the interdependence of science and technology with society. Gemstone students are members of a living-learning community comprised of fellow students, faculty and staff who work together to enrich the undergraduate experience. This community challenges and supports the students in the development of their research, teamwork, communication and leadership skills. In the fourth year, each team of students presents its research in the form of a thesis to experts, and the students complete the program with a citation and a tangible sense of accomplishment.”[53]
The educational impacts of Gen-2 ERCs included new degree programs derived from the ERCs’ research, including the following:
1. BPEC – MIT
The BPEC ERC at MIT had a broad impact on graduate and undergraduate engineering education at MIT. This impact would not have taken place without committed leadership from the ERC, demonstrating the persistence and patience needed to bring around MIT administrators to recognize the importance of molecular biology as a fundamental science underpinning the education of all engineers, just as physics and chemistry have been. The result was a broad institutional impact delivered by two efforts focused on bioengineering: a new department and a new Course 20, the first in 29 years at that time.
A Change in Perspective Prof. Doug Lauffenberger’s embrace of interdisciplinary research is especially remarkable because of some earlier history of involvement his with BPEC. While he was still a professor at the University of Illinois, Urbana-Champaign, Preston invited Lauffenberger to serve as a site visitor for one of BPEC’s annual reviews. She briefed the team the night before the visit started about the goals and features of ERCs and their interdisciplinary character. The next morning, just as the team was to enter the briefing room to start the visit, he pulled her aside and said he didn’t want to continue to serve because he didn’t believe in interdisciplinary team research. She told him he had a job to do and he had to use the ERC review criteria, not his own. He could go in and do that job or go home. He agreed to serve under those conditions and wrote an excellent analysis of one of the thrusts. A few years later, Danny Wang called Preston and told her: “Guess who wants to be a faculty member in BPEC and come to MIT?” It was Lauffenberger, and the rest is history. |
It was during the second NSF-funded life-span of BPEC (1995–2004) that Douglas Lauffenberger and Linda Griffith led teams in developing education programs supporting these significant impacts on the culture of research and education at MIT. In 1998, building on the team culture that integrated molecular biology and biochemical engineering—established in the first BPEC by Danny Wang (ENG) and Harvey Lodish (BIO)—Lauffenberger led the movement to establish one of two cross-disciplinary departments at MIT, the Department of Biological Engineering, initially serving as its Chair and continuing to serve until 2019. This department was a critical force for bioengineering research and educational advances at MIT. Lauffenberger provided a culture where interdisciplinary research and education were enthusiastically encouraged and supported—not a common occurrence in academe in the 1990s. (See sidebar.)
Griffith’s initial involvement in BPEC when she arrived at MIT in 1991 as a junior faculty member, and later as a full Professor and BPEC’s Director, brought increased innovation in bioengineering and tissue engineering research, as recognized by her MacArthur Fellowship (2006) and other awards. Because of her association with BPEC, she began to see a new role for bioengineering education at MIT. With this insight, she led a team of MIT faculty in the development of MIT’s first interdepartmental minor degree program in biomedical engineering in 1995, which became MIT’s most popular minor degree program. Her leadership of this interdisciplinary team of faculty resulted in the development of the undergraduate major in Biological Engineering, finally launched in 2005—the new Course 20. It was the first new undergraduate major at MIT in 29 years.[54] The result was that for the first time, undergraduate engineers at MIT had the opportunity to pursue degrees that integrated molecular biology and bioengineering knowledge to provide them with skills needed to advance research and technology in this growing field.
2. University of Colorado-Boulder and Engineering Education
The Ailes study indicated that the University of Colorado College of Engineering administration noted in 1998 that it had been working for the previous five years to change the education it provides to undergraduate students. This objective was said to have been spurred, in part, by the national debate on this issue and in part by the sense in the College of Engineering that students both need and want hands-on experience, increased communication skills, and greater concern for ethics and humanities. NSF’s efforts to promote similar themes were also considered a factor in making the administration re-think its education-related initiatives.
Within this context, the University of Colorado created an Integrated Teaching and Learning Laboratory (ITL). While the ITL was being developed, the Optoelectronic Computing Systems ERC (OCS) was running its own education programs that involved similar laboratory-type experiences for undergraduates. Cross-fertilization of ideas between the OCS and the administration worked to the benefit of both the ITL and the Center. The ITL’s development was independent of the ERC, but the ERC helped it along. The University was in the process of establishing a Discovery Learning Center (DLC) as an adjunct to the ITL at the time of the SRI/Ailes interviews, and the Center’s experience with undergraduate research teams was thought to have had more of a direct impact on the DLC than it had on the ITL, which was established at an earlier stage of the Center’s existence.[55]
3. DSSC – Carnegie Mellon University
The Ailes study averred that the Data Storage Systems Center (DSSC) (Class of 1990) was “seen as a major catalyst in the revamping of the (CMU) Department of Electrical and Computer Engineering’s (ECE) undergraduate curriculum.” This is an overstatement because, while DSSC was supportive of this change, it was already underway (involving considerable effort on the part of department leadership) and would not have been possible without the unanimous support of the ECE faculty more broadly. The report went on to say that “this curricular change was adopted throughout the College of Engineering and even, by some accounts, by other universities.”[56] This impact on electrical engineering education across the country was largely due to the leadership of Steven Director, a prior member of both the design-focused ERC at CMU and the DSSC, at that time the ECE department chair and later Dean of Engineering at CMU. He decided to undertake this innovation as a demonstration model for other departments of electrical engineering across the country, much like the USDA selects demonstration farmers to pioneer agricultural innovations. For these innovations, he was awarded the 1998 IEEE Education Medal “for contributions to electrical engineering education through innovative textbooks, leadership in undergraduate curriculum reform and inspired graduate teaching.”[57]
4. PRC – Georgia Tech
Gen-2 ERCs had stronger engineered systems and more experience with proof-of-concept testbeds than Gen-1 ERCs did, a fact which had a lasting impact on their education programs and their home universities. An example of this type of Gen-2 ERC educational experience can be found at the Packaging Research Center at George Tech. As shown in Figure 10-10, the PRC enhanced their students’ experience through the Design-Build-Operate (DBO) courses that gave them hands-on experience in electronics packaging in collaboration with industry. One of the DBO facilities is depicted in Figure 10-11, along with the course offered through it. The DBO was paid for with funds from the ERC Program, industry, and the Georgia Research Alliance, a statewide effort by Georgia to develop and implement policies to promote science- and technology-based economic development.
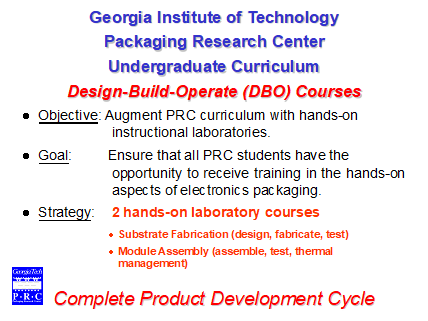
Figure 10-10: PRC’s Educational Curriculum – Theory to Hands-on Design/Build
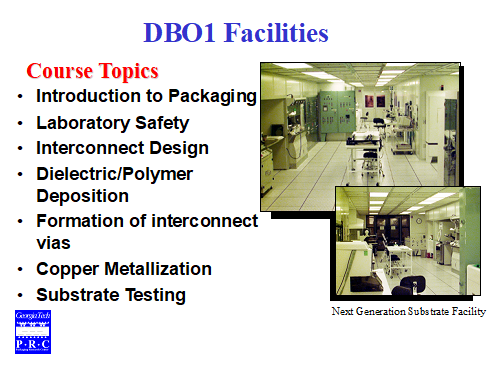
Figure 10-11: The PRC Design-Build-Operate Facility
5. CASA ERC – University of Massachusetts-Amherst
The CASA ERC was funded in 2003 as a multi-institutional ERC, headquartered at the University of Massachusetts (UMass), in partnership with Colorado State University (CSU), the University of Delaware (UD), the University of Oklahoma (OU), and the University of Puerto Rico, Mayaguez (UPRM). As such, it is one of the best examples of the type of broad-based cultural impact a Gen-2 multi-university ERC could have.[58]
The CASA ERC Director, David McLaughlin, understood the importance of proof-of-concept testbeds and decided to “allow” CASA students at UPRM to design and develop their own testbed. The only supervision was by a graduate student from CASA at UMass who had been in industry. The UPRM-CASA testbed was designed, implemented, and led by UPRM CASA students. They assembled a network of off-the-grid (OTG) radars that was capable of operating wirelessly using its own solar power sources and radars for advanced weather monitoring in a mountainous area of western Puerto Rico. The OTG network was tested in 2010. It proved itself more accurate than the conventional network when the student network succeeded in imaging a severe windstorm that was on a path to impact the Central American and Caribbean Games, which could have caused significant damage. Data from their radars were transmitted to the National Weather Service office in San Juan, allowing earlier warnings to be issued and the Games to be delayed until the threat had passed. The test successfully demonstrated a faster, more reliable system that can be used in emergencies and parts of the world where access to consistent power sources is unreliable.
CASA also developed a collaborative Ph.D. program between UMass and UPRM through which graduate students could go to UMass or CSU to take graduate courses and start their research, and then return to Puerto Rico to continue the research work at UPRM and finish their doctoral degrees in electrical and computer engineering. This program has resulted in the installation of young faculty at UPRM and SUNY Albany with the CASA and ERC experience.
CASA strengthened the M.S. in Professional Meteorology Program at OU by enabling students to take five core courses in meteorology, three electives, and four classes in a specialization area to complete the degree. The emphasis was on preparing students to more effectively communicate with customers about issues arising from storms and/or weather prediction. Unfortunately, the professor who was championing the degree program lost interest and it was cancelled, much to the dismay of many students.
The University of Delaware’s Disaster Research Center, an outreach partner in CASA, created M.S. and Ph.D. degree programs in Disaster Science and Management that were launched in 2010. Havidan Rodriguez, a key faculty member involved in the development of these programs, was a CASA partner and his experience with CASA and the interaction of social scientists, meteorologists and engineers in the development and deployment of the CASA radars impacted the way he developed these programs. CASA is used in the courses as an illustration of the opportunities and challenges of multi-disciplinary work. This course enables social scientists to gain deeper knowledge of engineering and meteorology and also enables students pursing engineering and meteorology degrees to gain deeper knowledge of social science and how public policy and technological innovation are interdependent in disaster management.
CASA and UMass benefitted by the appointment in 2004 of Dr. Michael Zink to CASA, where he served as the Deputy Director and contributed his systems experiences, gained through studies at German universities, to UMass engineering education through the development of new courses. These courses are characterized by integrative complexity and by the connectivity of social, economic, and political issues arising in the research creation and deployment of new technologies to solve societal problems. Raytheon, a CASA industrial partner, and UMass signed an agreement to make Raytheon’s Principles of Systems Engineering course a part of the curriculum of the College of Engineering.
v. Impact of ERC Education Directors Beyond their ERCs
One of the lasting impacts that ERCs have on the culture of engineering education is seen in the roles that ERC Education Directors play in their universities after their ERCs have graduated from NSF support. They often rise in the university system to bring the ERC approach to engineering education to the department or engineering school levels. A number of examples are given in Chapter 7, Section 7-E(a) in profiles of Martha Absher (ECT-Duke), Anne Donnelly (PRC-U Florida), Gary May and Leyla Conrad (PRC-GA Tech), Gigi Ragusa (BMES-USC), Penny Jeffrey (FREEDM-NCSU), and Beth Tranter (CPES-Va Tech). See also the more extensive discussion of the impacts of ERCs on engineering education in the following section 10-C.
vi. ERC Pre-College Educational Impacts
The Research Experiences for Teachers (RET) Program in ERCs (see Chapter 7, Section 7-D(b)) had broad cultural impacts on pre-college education because it brought teachers into ERCs to learn engineering concepts and returned them their classrooms with innovative engineering course modules, experiments, and experiences. However, it seems that sustaining an RET activity by ERC faculty after the ERC graduated from NSF support could only be achieved by winning an RET award from the NSF/ENG RET Program, because there was no obvious commitment to the concept at the department and engineering school levels. In 2018 Preston emailed the directors of graduated ERCs, asking if their ERCs had received RET awards post-graduation and if their departments or schools of engineering had picked up the RET idea and sponsored their own RETs. The returned responses came only from graduated ERCs that had received new NSF-funded RET awards, leading to the assumption that there was no ripple effect from an ERC’s RET programs on associated departments or schools of engineering, at least not sufficient to support internal funding of the program. The following ERCs received RET awards post-graduation:
- Microsystems Packaging Research Center (PRC), Georgia Institute of Technology, Class of 1994
- Engineering Research Center for Advanced Engineering of Fibers and Films (CAEFF), Class of 1998
- Extreme Ultraviolet Science and Technology Engineering Research Center (EUV), Colorado State University, Class of 2003
- Engineering Research Center for Integrated Access Networks (CIAN), University of Arizona, Class of 2008
- Engineering Research Center for Biorenewable Chemicals (CBiRC), Iowa State University, Class of 2008
- Engineering Research Center for Biomimetic MicroElectronic Systems (BMES), University of Southern California, (Class of 2003).
vii. Impact on University/Industry Partnerships
As shown in Chapter 6, “Industrial Collaboration and Advancing Technology,” ERCs undertook a broad-based “assault” on the engineering culture of the 1980s in academe. From an industrial-collaboration perspective, the culture that existed before the ERCs can be characterized as basic engineering research, largely supported by the government, or industry support for problem-solving applied research conducted by faculty researchers. The impact of ERCs was to establish a new culture wherein collaboration with industry in research ranging from fundamentals to systems and in the education of a more effective industry-oriented engineer was expected and successful, with positive impacts in both academe and industry.
The many assessments of the role of industry in ERCs have pointed to the establishment of fruitful partnerships that strengthened the role of long-term technology-motivated research in academe, brought strategic research planning to engineering faculty, and prepared an engineering workforce that had not only depth in fundamentals but also experience with industrial practice and leadership in innovation. The framework for collaboration broadened from the individual to the center, with faculty engaged in collaborative team research with firms and firms engaged with a wide range of other firms in the industry value chain, focused on advancing knowledge and technology in their fields of interest. Some ERCs, like the DSSC at CMU, had a broader industry-wide and global impact on the industries supporting them (see the sidebar).
Data Storage Systems Center’s Global Industry-wide Impact In 1990, representatives of two of the industrial sponsors of the Data Storage Systems Center (DSSC), an ERC at Carnegie Mellon University, met with then-Center Director Mark Kryder to discuss the establishment of an industrial consortium in the area of data storage technology. This discussion led to the formation in 1991 of the National Storage Industry Consortium (NSIC), a collective that sponsors over $135M in research through 11 collaborative programs. Known today as the Information Storage Industry Consortium (INSIC—see insic.org), it is the research consortium for the worldwide information storage industry. INSIC membership consists of nearly seventy corporations, universities, and government organizations with common interests in the field of digital information storage. Corporate membership includes major information storage product manufacturers and companies from the storage industry infrastructure. |
Most ERC industrial membership programs began at the proposal stage, where the prospective center director and other members of the team recruited members. At least two ERCs were generated by industrial consortia. These were the ERC for Environmentally Benign Semiconductor Manufacturing at the University of Arizona and the Center for Compact and Efficient Fluid Power at the University of Minnesota. See the linked file “Industry-generated ERCs_Case Studies” for a detailed summary of how they were generated, awarded, and the impacts they made on their industries.
Center leaders moved from collecting funds from firms to support center research to strategically targeting firms along the value chain to ensure broader industrial input and impact. The idea of using a value chain to display industrial members of an ERC was suggested by Peter Keeling, the Industrial Collaboration and Innovation Director of the Center for Biorenewable Chemicals (CBiRC), at Iowa State University, Class of 2008. As shown in Figure 10-12, the value chain displays that ERC’s strategy for developing industrial partners, from biomass producers to product users. The concept was adopted by the ERC Program and all ERCs were thenceforth required to use the value chain as a strategic planning tool for industrial collaboration.
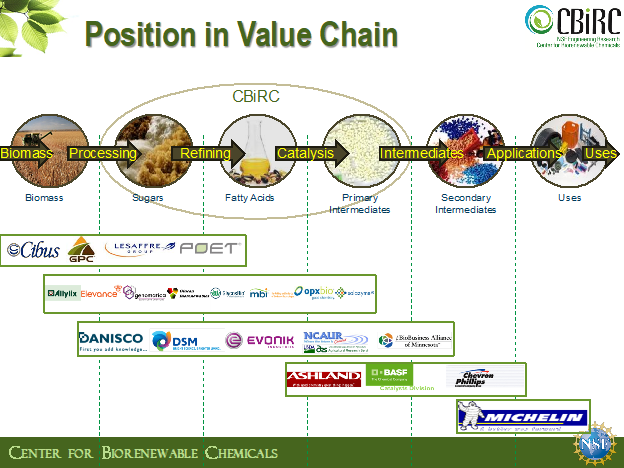
Figure 10-12: CBiRC Value Chain
10-B(b) Culture Change Brought About by Individual ERCs at Host Institutions
Several other ERCs also had important impacts on their department and university cultures—and often their local economies—but with less broad-reaching consequences than those above.
i. Entrepreneurial Education and Incubators (QoLT Foundry)
The Quality of Life Technology (QoLT) Center, an ERC at Carnegie Mellon University (CMU) was funded in 2006 to carry out research to advance robotic and other technologies needed to improve the quality of life of the aging and disabled. In 2008, the ERC began experimenting with a “Foundry” to identify and assist small start-up firms working to commercialize technology arising from the ERC’s research. The Foundry was an idea developed by Curt Stone, who served as the QoLT Industrial Liaison Officer and an Executive in Resident in CMU’s Office of the Vice President for Research. Curt had been a medical device entrepreneur for over 25 years before coming to CMU. Unfortunately, he died in 2012, but his legacy lives on at CMU.[59]
In contrast to the conventional ground-up approach of waiting for researchers to form start-up companies, the Foundry took proactive steps to deliberately create new QoLT-based companies. The goal of the Foundry was the creation of companies to market new-technology products that support older adults, people with disabilities, and/or their care providers. The Foundry was motivated by three drivers: (1) all three target populations express needs for such quality-of-life technologies; (2) many of those needs could be met quickly by researchers in and associated with the QoLT Center; and (3) regional economic development organizations were poised to facilitate commercialization of QoLT’s technologies. This aspect is one that was emphasized in all Gen-3 ERCs, which were established beginning in 2008.
Figure 10-13 illustrates the “process flow” of the Foundry. See the linked file “The QoLT Foundry” for a more detailed discussion of the development and operation of the Foundry.
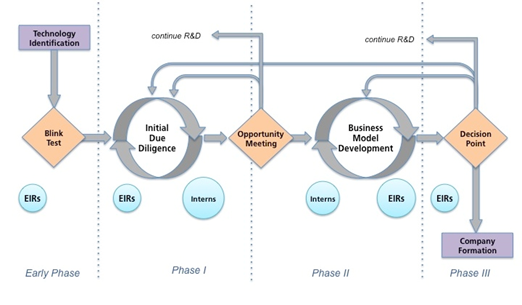
Figure 10-13: The QoLT Foundry Process (Credit: QoLT)[60]
ii. New Infrastructure
This section gives examples of how state governments used investments in academic infrastructure to support their visions for strengthened roles for university/industry interaction in research and education that could impact economic development and attract federal research funding. In some cases those investments resulted in an ERC being awarded to one or more universities in the state, further extending the realization of the state government’s vision. These entities serve as good models for other states, even today.
1. Centennial Campus at North Carolina State University
During the late 1970s and early 1980s, James B. Hunt, Jr., a visionary governor of North Carolina, planned for a new type of research culture where universities and industry could locate together to stimulate cross-sector collaboration to improve faculty knowledge of industrial practice, develop a new generation of students better versed in industrial practice, and speed the advancement of technology. In 1984, North Carolina State University launched on a path to fulfill this vision through the creation of the Centennial Campus. Governor Hunt was instrumental in the transfer of land by the State of North Carolina to NCSU. Hunt said, when reflecting in 2004 on the experience. “I’d been [thinking] so much about how to compete with the world, how we transform our economy, knowing that universities have to be the key to that, and in particular North Carolina State University. So I decided, ‘Listen, we ought to have a place where we can have business and universities and the best thinkers all working together, working alongside each other, parking in the same parking lots, having lunch together.’ The origins of the idea of Centennial Campus envisioning a place for students, faculty, industry, and government to live, learn, work, and play together were evident at this early stage of development.”[61] North Carolina responded to the need to join industry and academic research to advance technology and the technical workforce to strengthen U.S. industry through infrastructure, in a way similar to NSF’s role in strengthening the economy through the ERC Program.
After the development of a Master Plan under the direction of Claude McKinney, Dean of the School of Design, the first building was completed and occupied in 1989. Engineering played an important role in the development of the Centennial Campus. Larry K. Monteith, who was the Dean of Engineering at NCSU from 1974 to 1989 and served as the Chancellor from 1989-1998, helped bring Centennial Campus to life, and he was part of a group that helped give shape to the concept of the Research Triangle Park. McKinney noted in 1991 that “the Centennial Campus is a complimentary activity to the Research Triangle Park. In its park environment the Research Triangle facilitates research enterprises, which support individual corporate and governmental cultures. The Centennial Campus seeks to build a mixed-use, urban scale interactive environment which will facilitate collaboration between university researchers and corporate/governmental scientists.”[62]
This vision for the Centennial Campus was one factor that contributed to the awarding of the first ERC at NC State, in 1988—the Center for Advanced Electronic Materials Processing, led by Professor Nino Masnari. Masnari led the ERC until 1996, when he was appointed Dean of the College of Engineering at NC State, a position he held from August 1996 through June 2006, when he returned to the faculty of the Department of Electrical and Computer Engineering. Masnari’s experience working with industry before and during his leadership of the ERC shaped his vision for the future of NC State Engineering and its role in the Centennial Campus.
During Masnari’s tenure as Dean, the College of Engineering experienced a new era of growth and accomplishment. In 1997, the College held the grand opening for the Engineering Graduate Research Center, now the Monteith Research Center, and established the Women in Engineering Program. With the passage of a bond referendum in November 2000, the College began its move to NC State’s Centennial Campus. Masnari oversaw the construction of the first two academic engineering buildings to be constructed at NC State since 1964 — Engineering Buildings I and II which opened in 2004 and 2005, respectively. An adjoining Constructed Facilities Laboratory opened at the same time as part of the engineering research complex. Together these two buildings provide research, education, and demonstration facilities for the College of Engineering to use in developing partnerships with industry and government. Among the many components in the main laboratory building initially were the Center for Advanced Electronic Materials Processing, Analytical Instrumentation Facility, Biomedical Microsensors Laboratory, Power Semiconductor Research Center, Microelectronics Systems Laboratory, manufacturing clean rooms and teleconference rooms. In November 2006, NC State’s College of Engineering named the main entrance to the engineering building cluster on Centennial Campus the Nino A. Masnari Engineering Gateway. This was in recognition of “his many contributions to educational and research program development at North Carolina State University and for (his) vision and leadership in moving the College of Engineering to Centennial Campus.”[63] “Funding was secured and construction began on Engineering Building III under Masnari’s leadership and it opened in 2010.”[64] Today the Centennial Campus includes more than 70 corporate and government partners and more than 75 NC State research centers, institutes and laboratories. See the Centennial Campus web site at https://centennial.ncsu.edu/ for a full description of this unique campus. Two of those centers are the FREEDM and ASSIST ERCs.[65]
2. Buildings Dedicated for ERCs
In the early years of the ERC Program, a few ERCs were fortunate enough to be housed in new buildings, funded largely by their states and universities. These buildings were designed to encourage interdisciplinary collaboration, including special spaces where students could build testbeds, either virtually or in physical reality. One of these was the headquarters building for the ERC for Computational Field Simulation at Mississippi State University (MSU), established in 1990. The mission of that ERC was to research the means and methods to reduce the time and cost, while increasing the fidelity and scope, of complex field simulations for engineering analysis and design. Areas of focus were the design of aerospace vehicles, missiles, submarines, surface ships, automobiles, and turbomachinery, using recognized leadership capability in computational field simulation, scientific visualization, numerical simulation, and high-performance computing. The Center’s $5 million, 44,000 sq. ft. building located on the MSU campus was designed to promote cross-disciplinary effort, helping to promote the synergistic research collaboration essential to the Center’s success. One of the attractors for faculty was space reserved for them and their students, funded by the ERC. The headquarters building had a video classroom, affording the use of video equipment in classroom settings and allowing the production of videotaped classes or live satellite transmission to remote sites as well as network-based instruction. The Center housed a desktop infrastructure of general-purpose workstations and high-performance servers for faculty and student use. An immersive CAVE-like virtual environment facility (consisting of four 10×9-foot projected stereoscopic display surfaces, motion tracking, and voice-recognition) provided virtual reality research capabilities that were quite advanced in 1990. High-performance computing needs were served by a then-new supercomputer-class “supercluster” with supercomputers, infinite-reality graphics servers, and numerous workstations. The Center was also a heavy user of supercomputers at other installations around the country. In addition, the ERC was given dedicated space at the NASA-Stennis Space Center on the Mississippi Gulf Coast.
3. Investment in ERC Laboratory Infrastructure
In order to achieve engineered-systems proof-of-concept testbeds, some ERCs had to build new laboratory infrastructure. Funds for this type of large testing infrastructure largely came from university cost sharing, state funding, and industry support because NSF’s ERC budget was not large enough to fund the buildings to house them, the purchase or construction of the testing equipment, and maintenance costs. For other ERCs where proof-of-concept testbeds were at a smaller scale and the costs were not as large, NSF and other funds were used. Examples of the larger testbed facilities and their impacts on research and their fields follow.
Large Structural Systems. John Fisher, the leader of a team from Lehigh University, proposed the following vision for an ERC, entitled the Center for Advanced Technology for Large Structural Systems (ATLSS), to NSF in 1986:
Structures of highly efficient design will be fabricated of modular components made of composites of steel, concrete and many other materials. Design considerations will include ease of fabrication and construction. Provisions for monitoring and inspection will be built into a new structure. Automation and robotics will speed up many labor intensive processes. Life cycle cost, not first cost, will govern material selection. Computer design and control will be pervasive. Knowledge-based expert systems will assist in the design of both structures and details. Computers will facilitate rapid redesign, guide materials selection and fabrication, schedule erection sequences, and route the flow of resources used in fabrication and construction.[66]
To achieve that vision the ATLSS team proposed a large-scale experimental facility. “Inherent with ATLSS has been the need to study large-scale structures as multi-axis structural systems, and one goal in our 1986 plans was to construct a new stiff reaction-wall, strong-floor laboratory providing the capability to do multiaxis experimentation on full-scale structures. The fruition of this goal was the ATLSS Multidirectional Experimental Laboratory, which has a reaction wall wrapping around an end and one side of a 40-foot-wide by 100-foot-long strong floor (12m x 30m). The wall is 50 feet (15m) tall at the end and, along the side, incrementally steps down to 20-feet (6m) tall. Opened in 1989, it allows experimentation on full-scale assemblies up to five stories tall using a broad complement of computer-controlled hydraulic actuators to impose static, fatigue, and dynamic multi-axis loads. This laboratory, which is world renowned, is the physical cornerstone of the ATLSS Center, the heart of its research on large structural systems, and the testbed for other developments and innovations arising from ATLSS research.”[67]
Working with industry and government agencies, this facility enabled researchers to make the following advances:
- Compositions and the heat treatments needed to produce high-performance steels in the U.S.; ATLSS also developed parameters that enable these steels to be fabricated economically with or without minimal pre- and post-heating and with undermatching as well as matching strength weld metal.
- Innovative designs for structures that enable full utilization of the high-performance characteristics of these materials.
- Demonstration of the suitability of advanced, high-performance fiber composite materials as strength-enhancing materials, both when used with concrete and steel and when used independently in structures.
- A patented ATLSS Connector design, which facilitated field erection of steel frames, with prototypes installed in industrial buildings at two sites nationally and in industry in Australia.[68]
In the aftermath of the 1994 Northridge earthquake, the Center became the national focal point for the evaluation of new and retrofit designs of beam-to-column connections for welded steel moment-resisting frames (SMRF) and for the evaluation of welding procedures for SMRF.
ATLSS, in collaboration with the Navy and the shipping industry, also completed fundamental research into replacing the conventional surface-ship hull design with an innovative unidirectional double-hull design. Double hulls provide greater survivability and affordability for surface combatants, and greater protection against penetration and spills for commercial tankers. ATLSS affirmed this new concept through extensive theoretical and experimental studies into double-hull design, materials, fabrication and manufacturing systems, and serviceability. In addition, ATLSS coordinated a national Advanced Double Hull Technical Symposium, sponsored by the Maritime Administration and the Office of Naval Research and held in October 1994 at NIST.[69]
At the time of writing of the ERC’s final report in 1998, ATLSS had a “total research area (offices and laboratories) of about 50,000 sq. ft (4645 sq m) in the Imbt Laboratories building. The total cost of constructing, equipping and purchasing these facilities has been about $18M. Additional (funding) has been invested in upgrading the structural facilities in Fritz Engineering Laboratory, which are managed by ATLSS. Of this, about $1.8M was allocated from the ERC grant. The remaining 90 percent was contributed by Lehigh University (about $9M), the Commonwealth of Pennsylvania ($2M), and industry (about $5M).”[70] Forty years after its initiation as an NSF ERC, ATLSS remains a functioning cross-disciplinary center with research spanning fundamentals to proof-of-concept in the Fritz Engineering Laboratory and many other structural engineering experimental facilities on the Lehigh campus.
OTRC Wave Basin. The Offshore Technology Research Center (OTRC) was established in 1988 by the National Science Foundation as an Engineering Research Center, headquartered at Texas A&M University in partnership with the University of Texas at Austin. The vision was to increase the reliability and safety of deepwater offshore drilling platforms in order to enable economical exploitation of deep ocean resources. In the late 1980s drilling was feasible at depths of under 1500 feet; it required a 10-year research and development effort by industry, universities, and research organizations worldwide to enable drilling to 3000 feet or more.[71]
At the time the ERC was established, the deepest drilling depth was 1,350 feet, with steel jacket platforms. It was clear to the team that developed the ERC that this type of platform would not allow oil drilling in 3,000 feet of water or deeper. To be able to drill at these depths, because of the waves and the need to remain at these depths for a long period of time, a large floating body tethered to the bottom or anchored with taut or catenary mooring lines was needed in order to evolve from steel jackets to compliant structures (tension leg platforms, spars, etc.) and then to floating moored systems.
To attempt to achieve this type of technology, the OTRC undertook a research program, in collaboration with industry, to explore the feasibility of model tension leg platforms designed for 3,000 feet of water. This required theoretical studies of hydrodynamics and fluid structure interactions, the integrity of materials and structures, and seafloor/structures interactions. However, to achieve the technology goal, testbeds were required to validate the new design ideas experimentally in a 3D wave-generating facility—a wave basin—that provides 3-D waves, current, and wind interactions to develop a new nonlinear 3-D modeling “culture” and to test tension leg and compliant platforms at various scaling factors. The wave basin facility was built by 1991 with funds provided by Texas A&M University and the State of Texas. A three-dimensional wave maker, along with wind and current generators, simulates the conditions facing deepwater structures. The facility is 150 by 100 ft. in plan, with a depth of 20 ft. and a central pit, 30 by 15 ft. and 55 ft. deep, which allows modeling of complete vertically tethered structures in 3,000 to 5,500 ft. of water at scales of 1:55 to 1:100, without the need to truncate any of their elements. The structures can be subjected to the combined action of waves, current, and wind acting at different angles. The wave generator has 48 independently controlled paddles which can generate any specified time and spatial variation of the waves.[72] This facility continues to serve the research and industrial communities and has tested models of structures ranging from Tension Leg Platforms and Spars to Remotely Operated Vehicles for the petroleum industry as well as an Assured Crew Return Vehicle designed by NASA for the international space station.[73] OTRC’s industrial sponsors in 2003 are shown in Figure 10-14.
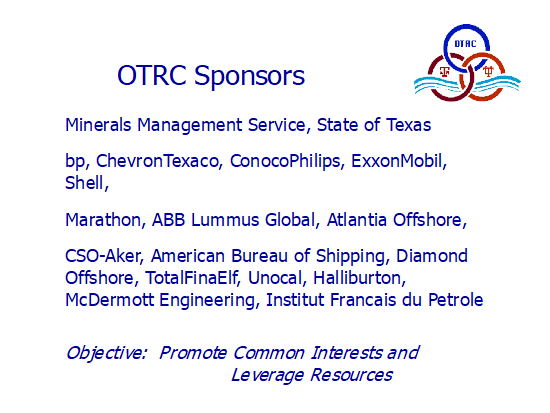
Figure 10-14: Sponsors/partners of the Offshore Technology Research Center in 2003
The Center for Biofilm Engineering (CBE), Class of 1990, at Montana State University maintains a 2,000 sq. ft. Prokaryotic Imaging Facility centered around its two state-of-the-art Confocal Scanning Laser microscopes, a 2,500 sq. ft. Surface Analysis Facility centered around CBE’s TOF-SIMS equipment, and a 2,000 sq. ft. Biofilm Systems Training lab. These facilities are extensively used by the Center’s Industrial Associates; workshops held in them to train industrial colleagues are well attended; and a large proportion of the “antibacterial” biomaterials that have been produced for use in medical devices are tested using the Center’s facilities.
The Packaging Research Center (PRC), Class of 1995), at Georgia Tech supports approximately 40,000 square feet of specialized facilities, including class 100, 1,000 and 10,000 clean rooms, and equipment for fundamental research, cross-discipline integration research, testbed fabrication research, and prototyping of microelectronic systems packaging technologies based on the Center’s System-on-Package (SOP) vision. These state-of-the-art facilities, valued in excess of $56M, are available to students, faculty, staff, visiting industry engineers, visiting scientists and scholars, and global collaborators to support the PRC’s research, education, and industry collaboration mission.
The Particle Engineering Research Center (PERC), Class of 1995, at the University of Florida is housed in a 25,000 sq. ft. building which includes the Characterization Research Instrumentation and Testbed (CRIT), a 17,000 sq. ft. facility for particle analysis, processing, and characterization. The facility includes six analytical laboratories and a high bay area and is available to ERC team members and other researchers from the UF and industry. Industry members may use the equipment and facilities for sponsored or collaborative research projects that fall within the scope of the ERC’s mission. Analytical services are available to industry partners at reduced rates and to non-industry partners on a time-available basis. The building was funded by the State of Florida with an investment of $5.3 million and completed in September, 1998. A clean room (class 10,000) has been created for research use. Between 1994 and 2000, over $4M has been invested in equipping and furnishing the CRIT, providing state of the art capabilities for conducting research and characterization of particulate systems.
The Pacific Earthquake Engineering Research Center (Class of 1997), a joint ERC among UC-Berkeley and eight partner universities, maintains experimental facilities including the nation’s largest geotechnical centrifuge, the largest three-dimensional shake table, and the strongest reaction wall/strong floor test facilities currently operating in the United States.
The Mid-America Earthquake (MAE) Center (Class of 1997), a joint ERC among the University of Illinois and six partner universities, maintains and monitors a network of ground motion instrumentation in the New Madrid seismic zone, providing an invaluable stream of real data to researchers. The facilities of the seven universities also include shake tables, reaction walls, centrifuges, and mobile test facilities for geotechnical engineering. The existing facilities at the MAE Center are available to those outside the Center when not in use. The estimated value of the existing infrastructure between the seven partner universities is on the order of $35 million.
The ERC for the Engineering of Living Tissues (GTEC), Class of 1998, was funded as a joint ERC at Georgia Tech and Emory University. it was headquartered in the Georgia Tech Parker H. Petit Institute for Bioengineering and Bioscience (IBB).[74] It was supported by a new, $30 million facility which includes an additional $1.5M, 7,000 sq. ft. vivarium. Many additional important core facilities are available within IBB. The ERC built a strong relationship with the Yerkes Regional Primate Research Center in Atlanta, a relationship that allowed the testing of tissue models, when appropriate, in primate hosts. Much work took place at the non-clinical research laboratories within both the Emory School of Medicine and the Veterans Administration Hospital and at operating suites at Emory University Hospital. GTEC facilities within IBB were shared by all faculty and students within IBB, both GTEC and others, and GTEC personnel share use of Emory facilities with other researchers throughout the Emory community. GTEC spent approximately $3.6M on equipment and facilities during the first three years of the ERC funding. These expenditures included the vivarium, flow cytometer, gamma counter, Beckman Coulter multisizer, microplate reader, sequence detector, CCD Imaging System, histology equipment, confocal microscope, and many other pieces of equipment purchased both for the Georgia Tech labs and the Emory labs.
4. Investment in Large Testbeds in an ERC and the Impact on Technology Transfer
Iqbal Hussain, the Director of the ERC for Future Renewable Electric Energy Delivery and Management (FREEDM) Systems at North Carolina State University, contributed to this History in 2018 the following observations about the power of testbeds in an ERC:[75]
The testbeds in an ERC in general drive the vision and mission of an ERC. At a more fundamental level, the testbeds are the platform for the development of the Enabling Technologies which lead to Systems development. In other words, the research agenda of ERC comes to completion with the help of the testbeds, and in parallel, center conceived innovations move towards maturity. The testbeds also play an important role in attracting the relevant industries to become members of the Center and provide appropriate and relevant mechanisms to train and educate the undergraduate and graduate students; testbeds and help further enhance the capabilities of the postdocs and other Faculty members. The development of the testbeds significantly facilitates the transfer of technology from a center to industry. In some cases, these testbeds enabled industry members to bring their components/devices for testing at an ERC.
The FREEDM ERC developed testbeds that significantly facilitated the transfer of technology from the ERC to the industry. Some examples provided by Husain follow:
“In several cases, these testbeds helped the industry members to bring their components/devices for testing in the FREEDM Center. The FREEDM Systems Center has three Large Testbed Systems:
- The first FREEDM Center Testbed is the entirely simulation-based Large-Scale System Simulation (LSSS) Testbed, which is quite flexible and was relatively lower cost to develop. This testbed allows evaluations of systems control algorithms and scalability. However, the limitation of an entirely simulation-based testbed is its inability to provide a platform for technology transfer evaluation or testing services for industry, although it is quite suitable to serve as a training platform for students.
- The second FREEDM testbed is the Hardware-in-the-Loop (HIL) testbed, which is ideal for a hardware component or real controller evaluation, with the rest of the system built in an emulator. FREEDM pioneered the approach for HIL-based testbeds for large systems that has the capability for technology transfer evaluation as well as for providing testing services to industries.
- The third FREEDM testbed is the Green Energy Hub (GEH) testbed, which is entirely hardware based, and hence is limited in scale and is expensive to fabricate and maintain. However, the testbed is the best option for prototype testing and evaluation, serving as the platform for testing services and workforce training. Several industries have utilized and continue to use this testbed for testing services. IEEE inverter interconnection standard conformity tests were also carried out in this testbed.
5. Industry-generated ERCs and Their Impact
By a decade into the life of the ERCs Program, the academic engineering culture had changed sufficiently that collaboration with individual corporations and industry groups directly in the formation of an on-campus ERC was possible. These were three-way partnerships between NSF, industry, and the lead university, involving ground-breaking negotiations and agreements. Two examples are described in case studies here and here.
10-C Impacts on Engineering Education
10-C(a) ERC Education Culture
Education is a central pillar of each ERC (see Chapter 7, “Education and Outreach Programs”). ERCs provide engineering students with wide-ranging cross-disciplinary research and education experiences. An ERC gives a student the opportunity to build technical depth, research complex systems, integrate knowledge from other fields to advance technology, lead and work in teams, and hone communication, innovation, and entrepreneurial skills—all prerequisites to effective engineering practice and creative and innovative leadership. An example is the CASA ERC student-run testbed (see sidebar).
Because of this culture, ERC students are highly sought after by industry and academe.[76] It has often been said that technology transfer is “a contact sport” involving person-to-person interaction. One of the main avenues for the transfer of ERC technologies to industry, resulting in products as described in the preceding section, has been through the ERC students that are hired by industry after graduation. They become the embodiment of the transference. Their ability to serve as agents of technology transfer derives not just from their exposure to new approaches to research but also from the novel education culture in ERCs. During their education these students are engaged in research projects with technology goals in sight—projects that require the integration of knowledge from more than one discipline and laboratory to reach completion, requiring a team approach among the faculty, students, and industrial collaborators.
Student-run Radar Testbed “Sees” Extreme Weather in Puerto Rico The Center for Collaborative Adaptive Sensing of the Atmosphere (CASA) was an ERC based at the University of Massachusetts-Amherst. The Center’s main thrust was (and still is) the development of networks of small, versatile radars that can “see” atmospheric phenomena such as tornadoes that occur in the lower regions of the atmosphere, below where current state-of-the-art Doppler radars can reliably detect. The aim is to detect such events sooner and in more detail. That capability has been proven in the field by experimentally deployed networks of the radars. One of these experimental networks, or testbeds, was deployed in conjunction with CASA partner the University of Puerto Rico–Mayaguez (UPRM). A team of CASA graduate and undergraduate students from several CASA partner institutions designed, assembled, and installed the “off-the-grid” (OTG), solar-powered and wireless radar system in a region of western Puerto Rico that the Doppler radar located south of San Juan cannot sense. The testbed covered a crucial 1.5 km-high gap in atmospheric weather-monitoring in that mountainous region, producing accurate rainfall data that could be used to predict flooding and support other applications such as crop hydrology. The testbed also explored methods for more accurate measurement of wind data than is now possible. It could be used as a back-up to the current radar system if the Puerto Rican electrical grid blacked out in heavy weather. In addition to its research value, the student-run testbed system offered a unique educational experience. It required the students to work independently as a group across disciplinary and geographical boundaries and to understand all facets of developing and implementing an experimental system in the field. The testbed provided the basis for development of a CASA course exploring recent weather radar research. In July 2010, the Central American and Caribbean Games at Mayaguez provided a real-world test of the power of the testbed. The CASA students were asked to support the games with their OTG radar network. The student network succeeded in imaging two damaging windstorms that impacted the Games, causing significant damage. Data from their radars were transmitted to the National Weather Service office in San Juan, which issued warnings early enough to delay the competition with minimal collateral damage. The test also addressed the tendency for conventional radars to report false alarms by debunking a false tornado report. This project not only has potentially big implications for the future of meteorology, but also was important for giving these engineering students real-world, hands-on experience in providing state-of-the-art technical services in an international setting with very little supervision. The students later gave presentations at the Weather Radar and Hydrology (WRaH) conference in England and the Institute of Electrical and Electronics Engineers (IEEE) Radar Conference in Kansas City, Missouri. |
The MIT Biotechnology Process Engineering Center (BPEC)—a member of the first class of ERCs and the only ERC to recompete successfully and complete its full second term as an ERC—is an example of an ERC that achieved that culture early, with significant impacts on its students, graduates, and member firms. The following anecdote exemplifies the type of student/industry interaction that was common across the ERCs.
Preston remembers attending a BPEC annual or renewal review at which a student talked about how the ERC’s culture enabled him to develop a new bioreactor design and then, instead of just writing up his dissertation, the collaboration between BPEC and an industrial member enabled him to travel to California and test how well his reactor worked in a real-world industrial setting. He would implement the reactor at the firm, gather data, and then return to BPEC weekly. This went on over a period of several months; it enabled him to perfect his design and the firm to complete a new process design in record time.
That student was Brian Kelley, an MIT Ph.D. graduate in 1992, who is now Senior Vice President at VIR Biotechnology; he was previously at Genetech and Tufts University. Dr. Kelley is a member of the National Academy of Engineering and his citation there points to the legacy of his BPEC experience: “For leadership in the development of bioprocess technology and cost-effective manufacturing processes for clinically effective recombinant protein therapeutics.” Dr. Kelley remarked to Preston in 2018:
My experiences at BPEC were an amazing exposure to many of the world’s experts in bioprocessing, covering cell culture, molecular biology, enzymology, microbiology, separations, and pilot plant operations. The critical mass of faculty and students that had been assembled at MIT was unparalleled and will forever influence the commercial industry and academic communities in the field of biopharmaceutical discovery and development.[77]
10-C(b) Education Outreach
A vital part of ERC education programs are outreach efforts to bring engineering concepts to pre-college classrooms, with the aim of attracting students to engineering and STEM careers. Dedicated senior faculty members and staff lead these university education and pre-college programs. Strategic planning of the education programs is an integral part of overall center planning and management. This practice helps to ensure the thorough integration of research and education that is a key feature of ERCs. The International Genetically Modified Machines (iGEM) competition, established in large part by the Synthetic Biology ERC (Synberc), is a case in point (see sidebar).
iGEM The International Genetically Modified Machines (iGEM) competition is an annual program in which undergraduate and precollege students learn how to build biological systems from standard, interchangeable parts. Organized by MIT with the support of the Synberc ERC, based at the University of California-Berkeley, iGEM student projects have steadily continued to grow in sophistication and reach. What started as a five-school design competition at MIT in the summer of 2004 had by 2018 grown into an international competition comprised of 344 teams from 38 countries and nearly 6000 students, faculty, and instructors. Teams use kits of standardized biological parts along with parts they design to build biological systems that accomplish a particular task. The teams travel to MIT to interact with other students and share their results in a juried, weekend-long summer competition. In the beginning, most projects were presented at iGEM at the design and modeling stage. Now, more projects are moving beyond that to also include building, testing, and measuring of the biological systems the teams develop. Projects now cover topics as varied as health and medical applications, biofuels and energy, environmental applications, and manufacturing. |
The centers have pioneered many innovative programs to leverage the strong aspects of ERC research and education not only into the engineering schools, but also out into the wider education system. An excellent example is the Research Experiences for Teachers (RET) in Engineering and Computer Science program, which was developed by ERCs and was the foundation for the Engineering-led NSF-wide RET Site program. The purpose of these programs is to bring K-12 science, technology, engineering, computer science, and mathematics (STEM) teachers and community college faculty to an ERC in the summer to work in the ERC’s laboratories to strengthen their knowledge of engineering and to return to their classroom with course materials that will bring engineering knowledge and constructs to their students. Each ERC is required to run its own RET program and is strongly encouraged to also compete in the broader RET Site Program competition.
In addition, each ERC offers outreach programs to involve talented high school students in the ERC’s labs, especially through the research-focused ERC Young Scholars Program.
Each ERC also runs its own Research Experiences for Undergraduates (REU) program to engage students from non-ERC universities and colleges in ERC research activities during the summer, working alongside ERC researchers and students.
Bringing greater diversity and broadening participation into the engineering workforce is an imperative for the continuing strength of the U.S. economy. Each ERC has a strategic plan for developing a climate of inclusion and success for all students—pre-college as well as university—through special outreach efforts focused on attracting and retaining diverse students to engineering and using the ERC culture to improve their retention in engineering. Every center has a faculty or staff member who is responsible for its diversity programs. See, for example, the essay provided by Claire Duggan, of the CenSSIS ERC.
US Science and Engineering Festival The “Powering Today and Tomorrow” exhibit at the 2014 US Science and Engineering Festival in Washington, DC, helped K-12 students learn about the transmission of electricity through everyday examples. Young students explored the behavior of static electricity generated by a plasma ball, the effectiveness of solar powered model cars, and the design of circuits that are similar to those found in homes. Over the three-day Festival, the ERC for Ultra-wide-area Resilient Electrical Energy Transmissions Networks (CURENT), headquartered at the University of Tennessee-Knoxville (UTK), saw several thousand student visitors. Helping with the exhibit were graduate students from UTK and teachers local to the university. Over 1,200 students visited the CURENT exhibit. |
The sections that follow provide specific examples of innovative engineering programs and courseware created by ERCs at the university and precollege levels, as well as programs aimed at improving the diversity of students and faculty involved with the ERCS and in engineering generally.
10-C(c) New Engineering Degrees, Courses, and Certificate Programs
ERCs have impacted engineering education at their host and partner institutions in many ways; but one of the most direct and lasting ways is through new curricula, up to and including new degree programs.
i. BPEC Biological Engineering
Early in the life of this ERC the need for a biology-based engineering curriculum was recognized that would prepare engineers to address future problems in biotechnology, pharmaceuticals, tissue engineering, and other areas.
The program began with a seminal course on therapeutic protein production, co-taught by Prof. Daniel Wang in engineering and Prof. Harvey Lodish in Biology. This course became enormously popular, and students asked for more subjects co-taught by engineers and biologists. Faculty responded by developing MIT’s first interdepartmental minor degree program for undergraduates—the Biomedical Engineering Minor Degree was initiated in 1995 and quickly grew to become the most popular undergraduate minor degree at MIT. More courses were developed, and in 1998 a new formal academic unit, the Division of Bioengineering & Environmental Health, was begun to foster develop of new academic programs at the interface of biology and engineering. The Division, soon re-christened “Biological Engineering” has faculty from Biology and from several engineering disciplines.
A new PhD program in Bioengineering began in 1998 and a Master’s of Engineering in Biomedical Engineering followed in 2000. In 2005, MIT established an entirely new course of study with the revolutionary Biological Engineering (BE) undergraduate degree program—the first new field of study at MIT in 29 years. As discussed above, BPEC’s Director, Linda Griffith, and former Director, Douglas Lauffenburger, were instrumental in shaping institutional consensus for the creation of the new degree program.
ii. SRC/ISR Masters in Systems Engineering
Under the guidance of its pioneering Executive Director, Ralph Schlenker, the Systems Research Center (SRC) at the University of Maryland and Harvard University (later renamed the Institute for Systems Research (ISR)—one of the original class of ERCs established in 1985—offered probably the most extensive and diverse education programs of any ERCs at that time.[78] A major vehicle for its impacts in education was an MS degree program in Systems Engineering (MSSE) established through the influence of the SRC.
Formally approved in 1990, this was a strategic, broad-based academic program that covered the gamut from systems definition, requirements, and specifications, through design, implementation, and operation, to the technical management of systems projects. The program consisted of required courses from systems engineering and management, electives from an area of specialization, as well as an optional thesis project demonstrating the practical implications of systems engineering principles while exposing students to the real world of systems engineering in the manufacturing sector. Participating faculty came not only from the universities, but also from industry and government agencies in the Washington, DC area.
iii. C-SOPS Masters in Pharmaceutical Engineering and Science
In 2009, Rutgers University won approval for its first new degree in a decade—a Masters of Engineering (M.Eng.) in Pharmaceutical Engineering and Science—in an effort pioneered by the Center for Structured Organic Particulate Systems (C-SOPS), an ERC based there. The degree embodies the concept of “relevant scholarship,” combining knowledge of the fundamentals and applications and preparing the students for success in either academia or industry. The degree program is targeted primarily at full-time industrial employees who earn their M.Eng. on a part-time basis.
The Center’s effort became the model for an Engineering-wide professional degree program at Rutgers, with each department within the School of Engineering being directed by the Dean to develop a similar program. The M.Eng degree is advertised to the Center’s industrial members, mentors, and sponsors as well as to pharmaceutical industry practitioners nationwide, in addition to current students.
The degree program was designed to provide graduates with skills and tools to be innovative, competent, contributing engineers in the pharmaceutical industry, with the ability to meet the constantly changing needs of industry for state-of-the art research/ manufacturing practices and protocols. Studies include five Pharmaceutical Engineering Core courses and a list of electives, several of which were new courses developed, approved, and taught during the 2008/09 academic year.
iv. Mississippi State’s Graduate Degrees in Computational Engineering
The Center for Computational Field Simulation (CCFS) was established as an ERC at Mississippi State University (MSU) in 1990 as part of the fifth cohort of ERCs. In 2001, the College of Engineering at MSU was comprised of five departments: electrical engineering, computer engineering, aeronautical engineering, computer science, and computational engineering. The computational engineering program was developed directly by the ERC.
When the CCFS started the computational engineering program, originally as an M.S. and then a full Ph.D. program actually administered by the Center, it was one of the first in the country. Getting the computational degree program established was considered by the University to be a significant accomplishment of the ERC.
v. Mississippi State’s M.S. of Fine Arts in Electronic Visual Imaging
The development of a Masters of Fine Arts in Electronic Visual Imaging at Mississippi State was also considered a direct spin-off of the CCFS ERC. It was reportedly one of the only programs in the country that involved collaboration between a College of Arts and Humanities and a College of Engineering. The artists and architects did not initially know about a type of virtual reality machine available in the CCFS until the Center brought them in and showed it to them. They immediately saw the utility of it for their own work, and the architects began doing virtual reality and the artists took a virtual trip to California with the use of a CCFS computer. Some on campus saw a drawback in the relationship of the graphic arts program to the ERC, however, because the program had been drawn too closely into the ERC rather than maintaining its independence. In addition, some in the ERC considered the graphic arts program a distraction because it did not contribute to the core mission of the ERC. Most, however, considered this linkage a major benefit to the University as a whole. It is believed that some of the first computer-graphic animators in Hollywood came from this CCFS experience.
vi. RMB Bioengineering Degree Program
In 2016, North Carolina A&T University (NCAT) became the first historically black college and university (HBCU) to attain full Accreditation Board for Engineering and Technology (ABET) accreditation for its Bachelor of Science program in Bioengineering. By one year later, NCAT was experiencing record enrollment and graduation of women and minority Bioengineering students. The program was initiated by the ERC for Revolutionizing Metallic Biomaterials (RMB), headquartered at NCAT.
NCAT now offers the first seamless undergraduate and graduate Bioengineering program at an HBCU. In Spring 2017, seventeen students were enrolled in the Master’s program and 95 students were enrolled in the Bachelors program, of whom 65% were female and 69% were African-American. Bioengineering is the application of engineering principles and techniques to the medical field. It combines the design and problem-solving skills of engineering with medical and biological sciences to improve healthcare diagnosis and treatment.
vii. CAEFF International Molecular Engineering Degree Program
The Center for Advanced Engineering Fibers and Films (CAEFF), an ERC at Clemson University (Class of 1998), headed an international team of faculty that developed an innovative undergraduate degree program in molecular engineering. (Molecular engineering entails the creation of molecules and the development of new products from them.) In October 2003, CAEFF hosted an invited workshop in Leeds, England, where the participants drafted an undergraduate curriculum for what was then a new field. Faculty from Clemson University, MIT, the New Jersey Institute of Technology, Lehigh University, McGill University, the University of Leeds, Cambridge University, Technion Institute of Technology (Israel), and the University of Bradford participated in the workshop.
The curriculum in molecular engineering blended physics, chemistry, biochemistry, advanced mathematics, and engineering. It was the first engineering discipline to design processes and products at the molecular level, enabling molecular engineers trained in the new curriculum to design from the molecular scale to the macro scale. Courses in the molecular engineering program were taught simultaneously via video-link at all participating universities. In most of the institutions, the curriculum was eventually subsumed under degree programs in biomedical engineering or bioengineering, although it does exist as a separate degree at some of these universities.
viii. VaNTH – CAPE and HPL
The VaNTH ERC for Bioengineering Educational Technologies (est. 1999), headquartered at Vanderbilt University, worked to develop learning tools for science and bioengineering. With the “Courseware Authoring and Packaging Environment” (CAPE), VaNTH created a tool that would help develop and review courseware based on advanced concepts in learning science like the “How People Learn” (HPL) framework.
VaNTH investigators sought methods for the development of courseware that drew from repositories of primary teaching content and organized these materials for delivery within a learning management system. They first identified a computing architecture for the delivery of instructional materials designed on HPL principles. Next, they developed a courseware authoring system that allowed instructors to assemble teaching elements (“granules”) into modules and higher-order course elements. They also created an experimental learning management system for delivery of these materials to students. Moreover, they extended the system to incorporate interactive and assessment elements.
This courseware authoring system and experimental learning management system were used to deliver modules in biomechanics, biotechnology, and bio-optics instruction. The system significantly reduced the time required for instructors to move from concept to implementation of courseware and provided the broad architectural framework for further development of VaNTH courseware. Of particular importance was the ability of CAPE to allow individualized instructional paths through material that can be designed to tutor students in the areas requiring most help.
ix. ASSIST Nanosystems Engineering Certificate
A new graduate certificate entitled Nanosystems Engineering was established in 2013 at North Carolina State University under the guidance of the Nanosystems ERC for Advanced Self-Powered Systems of Integrated Sensors and Technologies (ASSIST). An undergraduate minor in Nanoscience and Technology was also established to help motivate undergraduate students to enter the field as soon as possible. Two new courses specific to the emerging Nanosystems field were developed in support of these program offerings.
The new nanosystems program offerings are highly interdisciplinary and thus afford students opportunities for entry from multiple engineering pathways. The new courses combine several different engineering perspectives with practical entrepreneurship and innovation, so students can understand the opportunities and challenges in applying nanotechnology to developing new technologies and products or modifying current commercial projects. In addition to successfully completing technical courses, students are also required to complete two general education courses in topics such as diversity in the workplace, global issues in science and technology, and engineering ethics—requirements that enable well-rounded nanoscience graduates who can address our most urgent contemporary challenges.
x. CBiRC Biorenewable Chemicals Graduate Minor
In 2010, the Center for Biorenewable Chemicals (CBiRC), initiated a new Biorenewable Chemicals Graduate (BCG) Minor at CBiRC’s lead institute, Iowa State University. The BCG allows students from a variety of allied disciplines to understand the opportunities for developing biorenewable chemicals. Students in the minor gain explicit entrepreneurial internship experience, a background in the general issues related to production and processing of biorenewable resources, and exposure to the economic and environmental realities of the chemical industry. The educational experience combines high-quality technical education with hands-on entrepreneurial knowledge and skills.
The new minor consists of a 14 credit-hour sequence: 8 hours of graduate coursework in Fundamentals of Biorenewable Resources and Technology, Biological and Chemical Catalysis, The Evolving Chemical Industry, and a Biorenewable Chemicals Entrepreneurial Internship; as well as 6 credits of coursework selected from a list of courses reflecting CBiRC’s three technical thrust areas: New Biocatalysts for Pathway Engineering, Microbial Metabolic Engineering, and Chemical Catalyst Design.
xi. Synberc’s New Courses
The Synthetic Biology Engineering Research Center (Synberc) in 2009 launched a series of new courses in synthetic biology at the graduate, undergraduate, and high school levels. The courses reflect not just the scientific and engineering goals of the field, but also its social and ethical dimensions. Some of these courses were available through online modules at openwetware.org, and a course on the Fundamentals of Synthetic Biology was taught at the Korea Advanced Institute of Science and Technology (KAIST) in Daejeon, Korea.
Among the new courses SynBERC created are:
Synthetic Biology Lab: “SynBio Boot Camp.” A laboratory course designed as an introduction to research in synthetic biology. Students design and execute a real research project, and learn about methods and design concepts in synthetic biology.
Team Challenge: Molecular Design—Reading and Engineering the Recognition Code. A one-week intensive all-day class for first-year graduate students that combines experiments and computational simulation, in which teams of 4-5 students work towards solving a challenge question posed to the teams at the beginning of the week.
Genetic Devices. A graduate-level comprehensive survey of genetic devices. These DNA-based constructs are comprised of multiple biological “parts” that together encode a higher-level biological behavior and perform useful human-defined functions.
Biopower in the Contemporary Life Sciences. A mixed undergraduate- and graduate-level introduction to and discussion of questions of culture and power in the contemporary life sciences, with special attention to synthetic biology.
Ars Synthetica. A graduate-level collaborative seminar geared to the production of media for the Ars Synthetica Project, a Web-based multimedia forum for engaging specialists and non-specialists in an informed, ethical, and democratic dialogue on the emerging field of synthetic biology.
xii. CASA Networking Course
The ERC for Collaborative Adaptive Sensing of the Atmosphere (CASA) is based at the University of Massachusetts, Amherst but the impact of the Center’s work is felt far and wide. In Fall 2005, CASA professor Jim Kurose and research scientist Mike Zink led a team of nine undergraduate and graduate students in a course exploring recent weather radar research by developing, deploying, and measuring novel outdoor wireless networks based on the 802.11 wireless standard. Professor Kurose and three of the students offered a two-day version of the course at CASA partner University of Puerto Rico, Mayagüez. The course included lectures and hands-on outdoor installation and experimentation with wireless networks.
The initial course was archived in a multimedia format called jMANIC that provides a browser-based viewer of synchronized video, audio, and graphics with search and logging capabilities. A CASA student developed jMANIC as part of the CASA Education and Outreach thrust. The Puerto Rico two-day course was also archived and was made available on CD and on the Internet.
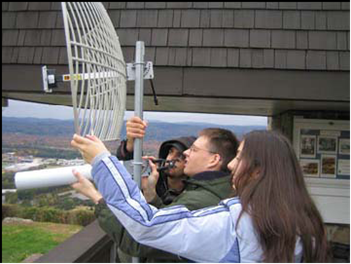
Figure 10-15: CASA students were heavily involved in setting up a radar network in Puerto Rico and Amherst. (Credit: CASA) |
CASA students helped to develop and deploy a wireless networking course at a partner university in Puerto Rico (Figure 10-15).
xiii. CISST Hands-on Surgery Course for Engineers
The Center for Computer-Integrated Surgical Systems and Technology (CISST), headquartered at the Johns Hopkins University, prepares engineering students to invent and develop advanced medical technologies. “Surgery for Engineers,” established in 2005, was a hands-on course designed for engineers tasked with the development of computer-integrated surgery tools that will improve upon current technologies in use in the Operating Room. The course taught engineering students the fundamental skills and operative procedures for general surgery. In doing so, it engaged students in new learning experiences, fostered relationships between engineers and clinicians, and enhanced the undergraduate curriculum for career preparation.
The impact of the course was at three levels: undergraduate, graduate, and in the medical field. Undergraduates got a hands-on laboratory experience unlike any other in their courses, challenging them to continue this experience into their research at the graduate level. At the graduate level, Surgery for Engineers provided a complimentary experience that often paralleled their current research projects, exposing them to the realities of surgery and further innovative ideas for surgical technology. The impact extended to the medical field, where engineers work with the medical staff to develop leading-edge instruments for use in the operating room.
xiv. BPEC Lab Fundamentals in Biological Engineering
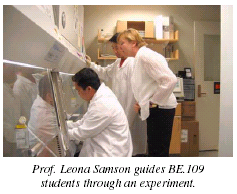
Figure 10-16: MIT’s BPEC has pioneered innovative courses in Biological Engineering (Credit: BPEC) |
The Biotechnology Process Engineering Center (BPEC) helped to found MIT’s Biological Engineering Division in 1998 (see section i above). BPEC leaders recognized that for biological engineering to become established alongside more traditional fields of engineering—mechanical, electrical, etc.—innovative pathways between biology and engineering were needed. The result was a new MIT course, “Laboratory Fundamentals in Biological Engineering” (BE.109), first offered in 2004. Originally taught by a BPEC faculty member (Figure 10-16) and still offered online in 2018, this course presents experimental techniques in biology and biochemistry from a quantitative engineering perspective, using the modern tools of genetic regulation to teach students to manipulate gene expression in cells. Three discovery-based experimental modules focus on RNA engineering, protein engineering, and cell-biomaterial engineering. The course acquaints students from more traditional fields of engineering with the concepts of biotechnology, preparing them for research as undergraduates or as graduate students in a Masters or Ph.D. program. The course is part of the curriculum of MIT’s new Course 20 in Biological Engineering (see section 10-B(a)iv).
xv. WIMS Graduate MEMS Micro-Machining Course
The University of Michigan’s Wireless Integrated MicroSystems (WIMS) ERC, established in 2000, viewed teaching microelectromechanical systems (MEMS) and microsystems technologies as being as critical for the future development of the field and the education of engineers as is research on new devices and technologies. The Center’s leaders considered comprehensive curriculum to be necessary at both the undergraduate and graduate levels. During its first five years as an ERC, WIMS developed and introduced a series of five courses on microsystems and MEMS. One of these, for example, was a Micro-Machining course (2003). This course covered emerging micro-machining technologies such as laser and electro-discharge machining; materials including SiC and diamond; and transduction techniques including electromagnetic, piezoelectric, resonant, and tunneling. The course discussed chemical and gas sensors, as well as sensors for biological applications. It also provided a review of microfluidics and its applications in different areas, including biomedical. Students carried out a team design project to develop, simulate, and design a device of their choice, and presented their findings in a technical article. The course used MEMS-specific CAD tools, such as Coventorware and ANSYS, that were cutting-edge at the time.
10-C(d) Precollege Engineering Teaching Modules
i. GTEC Biotech Modules
In 2008, the Institute for Bioengineering and Bioscience (IBB) at Georgia Tech received a $15,000 grant from the DeKalb County Development Authority to develop biotechnology toolboxes for use in local area schools. The program was developed in partnership with DeKalb County Office of Economic Development, the DeKalb County School System, and Georgia Bio. The IBB’s Biotechnology Education Toolboxes afforded Atlanta-area teachers the opportunity to share with their students hands-on laboratory experiences in cutting-edge techniques. Students benefit from this unique exposure to authentic, problem-solving approaches to real-world situations.
Development of these toolboxes was an outgrowth of the Georgia Tech/Emory Center (GTEC) for Tissue Engineering’s (an ERC) biotechnology middle school camp, ongoing since 2004, which provided 20 students each year with the opportunity to spend a week at IBB learning about the latest developments in bioengineering. Toolbox program development included three main elements: first, the biotechnology camp curriculum was translated into learning modules that could be used in any classroom and taught either as a week-long biotechnology camp or as individual lessons; second, IBB a pilot training program hosted in June 2008 with DeKalb County School teachers that trained them in the use of equipment and techniques so they could effectively utilize the toolboxes; and third, this core group of teachers then provided training to additional teachers to expand the reach of the program and implement wider use of the biotechnology toolboxes in classrooms.
Jackie Sullivan—An Engineering Outreach Crusader Jackie Sullivan is an associate dean of the College of Engineering and Applied Science at the University of Colorado at Boulder (CU). She is founding co-director of the award-winning Integrated Teaching and Learning Program, which is focused on integrating hands-on engineering throughout the K-16 learning experience for approximately 4,200 undergraduate and 2,000 K-12 students annually and is Co-Director of the multidisciplinary Engineering Plus Program at CU. She received her PhD in environmental health physics and aquatic toxicology from Purdue University and brought 14 years of industry engineering and leadership experience to the higher education environment. At CU, Dr. Sullivan initially served as the director of an interdisciplinary water resources and environmental engineering simulation and optimization research center, before changing her focus to the K-16 educational mission. Sullivan leads the multi-institutional initiative that created TeachEngineering—an NSF-funded online, searchable, standards-based, digital library collection of 900+ K-12 engineering lessons and activities. Many ERC pre-college education modules can be found on the site. Sullivan led the 2004 founding of ASEE’s K–12 and Precollege Engineering Division, served as a member of two recent NAE committees—”Engineering in K-12 Education” and “Changing the Conversation: Messages for Improving Public Understanding of Engineering”— and served on the Engineering Directorate Advisory Committee at NSF. She is a founding board member for the Denver Schools of Science and Technology—a network of highly-successful public middle and high schools that immerse diverse urban students in a liberal arts STEM-focused college preparatory education. She has published broadly, from Science magazine to NAE’s The Bridge. Dr. Sullivan received the 2005 Lifetime Achievement Award from the ASEE K-12 Engineering Division and the 2008 NAE Gordon Prize for Innovation in Engineering and Technology Education. |
Through 2009, funding from the DeKalb County Development Authority was used to develop three fully equipped biotechnology toolboxes and to train seven teachers in histology, gel electrophoresis techniques, and bacterial transformation. Activities, lesson plans, and problem-based learning questions were developed for classroom use, which were included on a resource CD with each toolbox. A set of training videos was also created and was added to the program’s website.
ii. Online Simulation Powered the “Youth Take Heart” Education Program
High school and middle school students often require an entertaining, multimedia approach to spark their initial curiosity about science and engineering. Once “hooked,” they can be enticed into further study of science and engineering as their exploration widens and they discover a fascination with various subjects. In 2003, the University of Washington Engineered Biomaterials (UWEB) ERC, in collaboration with the UW School of Art, developed a complex and entertaining online simulation aimed at young students. Called “Guy Simplant: The Case of the Ailing Heart,” it was a set of interactive games built around the concept of a “special agent” who is battling the forces of darkness in the form of an Evil Spy but who finds he is cardiovascularly impaired.
With $1.55M in backing from the National Institutes of Health, UWEB teamed with the Hope Heart Institute in collaboration with Seattle-area teachers to launch a health and science education program built around the Simplant game. The UW School of Art and the Washington Mathematics, Engineering, Science Achievement program (MESA) were also involved.
This initiative, called “Youth Take Heart,” was a five-year educational plan focused around cardiovascular health and aimed at students in grades 6–12. The effort had two main emphases. The first was to introduce students, especially minority students, to careers in health and science; and the second was to educate them about good health practices. In the process of helping Guy, students learned how the heart works, what can go wrong and why, and how heart disease is treated. They were asked to set up a program for Guy to manage his diet, exercise and stress, and they had an opportunity to see the results. At the same time, the students learned about tissue engineering and a cutting-edge research project by the UWEB and Hope Heart to grow living tissue to repair damaged hearts.
The Youth Take Heart program also included a laboratory kit and general curriculum. The program continued beyond the graduation of UWEB, with the Hope Heart continuing to offer it to students across Washington state. See http://www.hopeheart.org/projects/youth-take-heart-2/
iii. BioSIGHT—Interactive Visualization for Science Education
Beginning in 2001, the Integrated Media Systems Center (IMSC) at the University of Southern California collaborated with NASA’s Jet Propulsion Laboratory (JPL) on a project called BioSIGHT—Interactive Visualization for Science Education. This was an applied
WrestleBrainia WrestleBrainia 3000 (WB3000) is an engaging two-player game that utilizes electrical signals from muscles to allow users of all ages to play an arm-wrestling game where neuromuscular signal strength, instead of physical strength, determines the winner. Participants get direct visual feedback about their muscle firing patterns and learn basic concepts about the nervous system and neural engineering. WB3000 was developed by student researchers in a design competition judged by industry representatives at the Center for Sensorimotor Neural Engineering (CSNE), an ERC with headquarters at the University of Washington. More than 1000 people, from elementary school children to elderly adults, played WB3000 at several large events in the first eight weeks after it debuted in February 2013. WB3000, developed by CSNE students (the team’s leader was an undergraduate), helped showcase the talents of CSNE students and raise public awareness of the potential of neural engineering to create fun games, transform the human-computer interface, and provide life-changing assistance to people suffering the devastating effects of stroke, Parkinson’s disease, and spinal cord injury. Thousands more players tried the game over the next two years, before its developers moved on to develop and deploy a new neural engineering game. |
research project in the area of K–12 education that used BioSIGHT’s Interactive Streaming Storyboard (ISS) Tool, a software system for teachers to customize, deliver, and present multimedia content for their students, using visual learning as a pedagogical approach. The goal was to design, develop, and deploy tools and prototypes that could support and enrich K-12 teaching and learning with value-added applications of “immersive” technologies—an area of media in which the IMSC specialized.
The BioSIGHT-JPL collaboration used the ISS Tool, in conjunction with the rich multimedia content of the JPL, to develop space science curriculum for K-12 students. The project involved developing and prototyping multimedia modules of curriculum to research and document the impact of a technology-enhanced learning environment on students’ understandings of complex scientific models. The pedagogical strategies adopted include learning through play, learning with technology, as well as inquiry-based learning. The exploratory challenge introduced visual and spatial concepts as well as promoted dialogue and discussion among students and their teacher through a game-like simulation. The storyboards conveyed lessons through animated interactive storyboards and visual summary maps. The IMSC-JPL collaborative investigations extend the use of storyboards for deeper understanding through inquiry-based activities.
The collaborators first implemented the project using JPL’s contacts in 20 schools in 4 states in 2002. The initial project made the space science curriculum entitled “Ice Experience” available to inner city and rural Native American children. In addition to its educational and outreach benefits, the project allowed the IMSC to explore ways to incorporate BioSIGHT’s ISS Tool as a vehicle of collaboration between geographically distributed students, as well as find ways to implement the tool for teacher training in conveying innovative methods of science learning.
iv. VaNTH Modules
In their efforts to catalyze a more effective workforce in biomedical engineering, the VaNTH ERC sought to develop new educational methods and approaches at the precollege, undergraduate, graduate, and postgraduate levels. In 2003-2004, VaNTH’s work at the precollege level was especially productive.
The goal was to integrate biomedical engineering challenges into the science requirements for middle and high school students. Precollege teachers helped in the development of materials and teaching modules. The modules included the electrocardiogram; biomechanics through the study of balance and gymnastics; optics through the study of LASIK; fluid dynamics through a study of hemodynamics; and energy systems of the body through the study of swimming, metabolism, and medical imaging. These modules were developed in Nashville, Chicago, and Austin and implemented in both private and public schools.
Each module was analyzed statistically through the use of pre- and post-tests as compared to control classrooms. Three modules were shown to statistically improve student performance on basic science questions. Field-testing occurred in the 2002–2003 and 2003–2004 school years and a new module on fluid dynamics through a study of hemodynamics was added.
10-C(e) More Effective Engineering Graduates
Not only in bioengineering, but in every field of engineering touched by the ERCs—encompassing most of the emerging areas over the past 30+ years—the ultimate goal of ERC education programs has been to produce graduates who could lead in technology development in industry as well as in academic research.
Section 10-C(a) described the “ERC education culture” that contributed in a unique fashion to shaping that type of engineer. At MIT’s Biotechnology Process Engineering Center, for example, this education culture produced a whole new generation of bioengineers skilled in biology and engineering, who became the new engineering leaders in the then-emerging biotechnology industry. The following list presents a small sample of those early BPEC graduates and their impacts in industry, up to 1999.
Early Examples of BPEC Exemplar Graduates’ Accomplishments:[79]
Noubar Afeyan, Ph.D.—Founded and built a new company, PerSeptive Biosystems, Inc., to commercialize perfusion chromatography, a technique now used throughout the biopharmaceutical industry.
Rebecca Dabora, Ph.D.—Developed improved production strains for the manufacture of Lovastatin, precursor to Simvastatin (ZOCOR®) at Merck.
Matt Croughan, Ph.D.—Developed the first licensed, high-density, fed-batch cell culture process (for production of Pulmozyme™), the first process to enable economically viable production of a high -dose therapeutic protein from recombinant animal cells
Michael Thien, Ph.D.—Successfully led the Merck project team for Crixivan®, the first HIV-1 protease inhibitor and a key component of new breakthrough therapies for AIDS, and the first high-dose therapeutic with five chiral centers.
John Aunins, Ph.D.—Developed and/or enabled the commercial cell and virus culture processes for production of Merck’s Hepatitis A Virus (VAQTA) and Varicella Zoster Virus (chicken pox, VARIVAX) vaccines. Contributed to the identification of HIV-1 protease inhibitor (Crixivan®).
Mark Applegate, Ph.D. and Dawn Applegate, Ph.D.—Helped develop a unique, combined bioreactor, packaging, and cryopreservation system for the first large-scale production and distribution of a fragile, living, engineered tissue-construct for transplantation therapy.
Robert Kiss, Ph.D.—Developed the first large-scale barrier system for elimination of viral contamination in industrial cell culture operations.
David Volkin, Ph.D.—Developed improved formulations for live virus vaccines, including Merck’s Varicella Zoster Virus (VARIVAX) and trivalent measles-mumps-rubella vaccines (M-M-R®II).
Jeff Cleland, Ph.D.—Project team leader and early champion of novel slow-release systems for more effective delivery of therapeutic proteins.
Anna Hagen, Ph.D.—Played a critical role in development of the purification process for Merck’s Hepatitis A Virus vaccine VAQTA; winner of the first ACS Industrial Biotechnology Award.
The sidebar “BPEC Graduates Are Leaders in Biotech Industry” provides further elaboration on major advances achieved by BPEC graduates in the biopharmaceutical industry.
BPEC Graduates Are Leaders in Biotech Industry Throughout the 1980s and 1990s, BPEC cross-trained hundreds of students in engineering and biology. BPEC alumni now occupy positions of leadership in nearly every major biopharmaceutical company, such as Genentech, Amgen, and Biogen-IDEC, and also at many pharmaceutical companies, such as Merck, Wyeth, and Bristol Myers Squibb. BPEC graduates at Genentech, for instance, played key roles in bringing new cancer therapies to market. These new monoclonal antibodies, named Rituxan®, Herceptin®, and Avastin®, kill selected cancer cells without causing the broad-scale, systemic cell damage/death of traditional chemotherapy. BPEC alumni not only helped develop new high-titer, fed-batch manufacturing processes for these drugs; they also helped design and build new manufacturing plants in California, some of the largest and most automated plants of their type in the world. BPEC graduates at Merck, as another example, have played key roles in the global battle against AIDS. In the early 90’s, BPEC alumni at Merck manufactured parts of the AIDS virus as drug screening targets. Merck then tested their library of chemicals to see if any could disrupt the function of these virus targets. They found a promising candidate molecule, one that inhibited the activity of the HIV protease enzyme, but it was among the most difficult molecules that the pharmaceutical industry had ever attempted to synthesize. From this work emerged the current protease inhibitor drugs that are used to combat HIV. Several BPEC graduates, as members of the Merck process team, developed a new manufacturing process and played key roles in the design and start-up of a new manufacturing facility in Elkton, Virginia. The new drug, Crixivan®, when combined with older AIDS drugs, could clear the AIDS virus from the blood stream of many victims and allow them to lead productive lives. It is not a cure, however. Other BPEC alumni at Merck are working on new AIDS vaccines. Their recent success with many other vaccines, including ones for Hepatitis A and chicken pox, provide hope that we might one day have an effective AIDS vaccine. |
In Chapter 6 of this History, “Industrial Collaboration”, Section 6-G profiled a wide range of ERC students (and faculty) who had achieved notable success in industry across many different fields. These include a sizeable number of founders of high-tech companies, who based their entrepreneurship on their research and education at an ERC.
Individual programs at some ERCs have focused specifically on small-business generation by students. A notable one was the QoLT Foundry, at the Quality of Life Technologies ERC based at Carnegie Mellon University. The Foundry was described in detail earlier in this chapter in section 10-B(b).
Similarly, in 2010 the ERC for Mid-Infrared Technologies for Health and the Environment (MIRTHE), based at Princeton University, launched an Investment Focus Group (IFG) intended to bridge the gap between innovation and commercialization through conferences that brought the Center’s students and faculty together with venture capitalists on a regional and national level. The case study describes a company, Sentinel Photonics, that resulted from in part from the IFG and MIRTHE involvement.
_____________________________________________________________________________________________________
CASE STUDY
Sentinel Photonics
In 2008, recent Rice University Electrical and Computer Engineering PhD graduate Stephen So came to Princeton University as a post-doc in MIRTHE Professor Gerard Wysocki’s group. Along with a friend and colleague at Rice, in 2010 So founded a startup called Sentinel Photonics, focusing on high-efficiency laser-based gas sensors for use in sensor networks and portable devices for industrial, health, homeland security, and environmental sensing applications. Sentinel’s unique technology quickly attracted the attention of leading university research groups and national laboratories for use in scientific research.
After incorporation, Sentinel became an affiliate industrial member of MIRTHE and participated in meetings of its Investment Focus Group, as well as in summer workshops for industry put on by MIRTHE. The company also hired a MIRTHE post-doc working in the lab of Claire Gmachl, the director of the MIRTHE ERC, as well as an undergraduate who had previously been a MIRTHE REU (Research Experiences for Undergraduates) student to work part-time during the school year. Sentinel also benefitted from relationships with MIRTHE faculty advisers.
Looking back in 2012, So reflected on the challenges of starting a company and “trying to develop our own distinguishing technology” during the Great Recession. Investors were hard to find and sought only low-infrastructure projects (meaning mainly software). State and local business development funding in New Jersey had dried up, and banks were loaning money typically only for companies that had been in operation for more than two years.
So credits Sentinel’s experience as a MIRTHE affiliate with providing the company with networking opportunities, connections to vendors and customers, and access to specialized equipment. He was also able to make an Elevator Pitch presentation at the Princeton Keller Center Innovation Forum, which was judged by venture capitalists, and to represent Sentinel at a tech transfer showcase held at a major international conference. He recognizes that although Sentinel faced the same struggles as all other startups, nevertheless the connection with MIRTHE enabled him to start a company with a generally solid business foundation and later enjoy some advantages of being an industrial member of the ERC.[80]
In June 2016, Sentinel was sold to Aeris Technologies, a mid-infrared laser gas sensor company, where So is now the vice president. _______________________________________________________________________________________________________
10-C(f) Outreach for Diversity
Bringing greater diversity into the engineering workforce and broadening participation in engineering education and practice is an imperative for the continuing strength of the U.S. economy because broadening the pipeline brings greater numbers of talented people into the engineering workforce and because, in general, a diversity of backgrounds and perspectives can stimulate new ideas and innovations.
Although increasing the participation of underrepresented minorities and those with disabilities was not a major emphasis of the ERC Program at its outset, by the beginning of Gen-2, with the Class of 1994-95, ERC solicitations required such an emphasis and by 2004, ERCs were required to have a diversity strategic plan. Since then, each ERC has a strategic plan for developing a climate of inclusion and success for all students—pre-college as well as university—through special efforts focused on attracting and retaining diverse students to engineering and using the ERC culture to improve their retention in engineering. Every center has a faculty or staff member who is responsible for its diversity programs. In this section several representative examples of such programs from across a range of earlier and more recent ERCs are described.
Greg Carman, Director, TANMS ERC — A Personal Reflection When I became the director of TANMS in 2012, I believed that the U.S. had reached a reasonable level of racial and gender equality for everyone. But after serving in my capacity as director for a while, I realized that the U.S.—and, indeed, I myself—needed still more evolution to reach true equality. So I began to ask myself and others what steps I could take from my admittedly small position of influence to make a difference. Now I routinely tell departments that if they want to improve their national rankings, the easiest way to do it is to improve their diversity—i.e., diversity is the key to success because more different ideas from different perspectives produce more revolutionary results! The thing that most disturbs me is that now, in my (second) awakening, our country seems to be moving away from those goals and ideals. The optimistic side of me believes that this backward step is nothing more than a brief oscillatory motion (in the wrong direction) prior to making that next big push toward true equality for all! But underlying that on a personal level, what I want to share with readers of this History is that my involvement with this ERC has made me (and many others) more self-aware as well as a more active advocate for societal change to achieve equality across the board. That is a gift I did not expect to receive and one for which I am grateful. |
(See also Chapter 9, section 9-L, for a discussion of the evolution of the ERC policies regarding diversity, which began with data collection in 1985, became a requirement by 1994, and culminated in a requirement for a diversity strategic plan by 2004.)
i. Multimedia University Academy
The Multimedia University Academy (MUA) was a program pursued by the Integrated Media Systems Center (IMSC) at the University of Southern California (USC) for several years beginning in 1997. It provided an academic environment for at-risk students to receive training for entry-level positions in new media and computer-based companies. Offered as a 20-week certification program for students aged 18-21, the program’s goal was to train inner-city minority students in multimedia, computer, and college preparatory skills.
MUA training emphasized workplace skills and attitudes embedded in a problem-based, authentic-task curriculum. The Academy’s mission was to build self-motivation, self-confidence, and skills in at-risk youth so that they could be productive in a professional work environment or independently pursue a career path in engineering, computer sciences, or computer-related occupations. The MUA gave companies access to potential entry-level employees and did not charge a fee.
In the five-year period from 1997 to 2002, 95 students participated in five MUA sessions. Of these, 75 completed the training and 7 out of 10 graduated students were employed or were attending college on a full-time or part-time basis. In 2002, the MUA program was replicated and licensed, and began being offered as an off-campus program by a for-profit organization, the New Media University Academy. The training also continued for several years to be offered by USC.
ii. The DAPCEP Connection
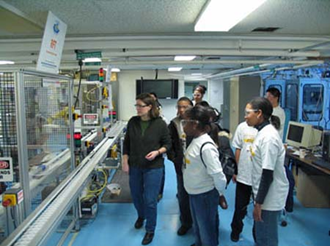
Figure 10-17: The DAPCEP program aims to interest inner-city minority students in engineering study. (Credit: RMS) |
For a number of years beginning in 2000, the University of Michigan (UM) ERC for Reconfigurable Manufacturing Systems (ERC-RMS) worked with the Detroit Area Pre-College Engineering Program (DAPCEP). The aim of DAPCEP—which still exists and which still has the UM as a partner—is to increase the number of historically underrepresented students who are motivated and prepared academically to complete a university curriculum in science and engineering. (See Figure 10-17.)
The ERC’s DAPCEP outreach program provided an opportunity for inner-city K-12 students to be exposed to various aspects of design and manufacturing engineering. Each year, between 30 and 40 middle school students (7th and 8th graders) from underrepresented groups in the Detroit area were brought to the ERC-RMS on Saturdays, over a 5-week period. As part of the ERC-RMS program, the students could choose between two programs: “Learn New Ways of Making Things” and “The Making of the Automobile.”
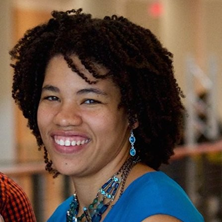
Figure 10-18: Dr. Michele Goe, a successful DAPCEP/ ERC-RMS graduate (Credit: RMS) |
Many of these students went on to pursue undergraduate degrees in engineering in high-ranking institutions, including the UM. For example, Michele Goe (Figure 10-18), who was a DAPCEP student, entered UM as a Mechanical Engineering major and received a GEM scholarship to pursue a Master’s degree in Operations Research in the ERC-RMS. Eventually earning a PhD in Materials Science, Dr. Goe is now (in 2019) the Assistant Vice President for Research at Acadian Asset Management, a global investment management company headquartered in the Boston area. She is but one example of the substantial impact the DAPCEP/ERC-RMS connection had on increasing the pool and diversity of students prepared to pursue an engineering degree at accredited institutions.
iii. Native American Students Shooting for the Stars Through Robotics
For a number of years, the ERC for Compact and Efficient Fluid Power (CCEFP), based at the University of Minnesota, sponsored an education outreach program called “Shooting for the Stars,” which enabled Native American students from the Ajibwe Tribe to discover robotics as both an after-school activity, which often included their fathers, and as an elective class in grades 2-12 at two schools, Albrook School and the Ojibwe School.
The CCEFP’s focus on Native American students, spearheaded by Education and Outreach Director Alyssa Burger, began when it sponsored a team of 20 high school students from the Fond du Lac Indian Reservation community in the Midwest regional FIRST Robotics competition, held at Williams Arena in the spring of 2008. The CCEFP-sponsored anishinaabeg ogichidaag (Ojibwe Warriors) FIRST Robotics Team was formed by students from a group of high schools near Cloquet, MN, and was the first-ever Native American FIRST team in Minnesota. In 2012, with CCEFP sponsorship, a secondary robotics program was launched at Cloquet Middle School.
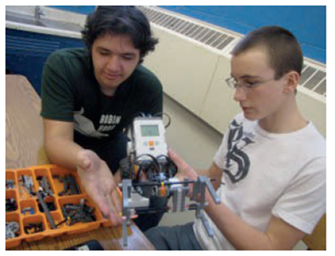
Figure 10-19: Native American students engaged with other K-12 students in building a robot through the CCEFP ERC’s Robotics Program. (Credit: CCEFP) |
The CCEFP’s Robotics Program (Figure 10-19) was part of a larger set of initiatives in northern Minnesota known as gidaa (gidakiimanaaniwigamig—”our earth lodge” in the Anishinaabe language) that are designed to engage area Native American students in active study as they work toward high school graduation and prepare for post-secondary education in STEM areas. Other K-12 gidaa activities sponsored by the ERC included science fairs and summer camps. CCEFP contributed to the programmatic, course content, and financial support of projects under the gidaa umbrella. Gidaa Robotics teachers drew on lessons learned through earlier gidaa experiences and introduced K-12 robotics day and after-school activities into their local schools using Lego Wedo-Webots and Lego Mindstorms robots and associated curricula.
iv. Linkage with LSAMP and AGEP
Two of the most substantial and longest-running programs at NSF aimed at improving the diversity of the science and engineering workforce are the Louis Stokes Alliance for Minority Participation (LSAMP) and the Alliances for Graduate Education and the Professoriate (AGEP).
The LSAMP program is a multidisciplinary program aimed at undergraduates interested in pursuing a career in science, engineering, technology, or mathematics. There are several LSAMP alliances nationwide, some regional in nature, such as the Pacific Northwest LSAMP, and others comprised of state universities such as those of the Texas and California state systems. Each LSAMP alliance consists of several academic institutions working together toward the goal of increasing the number of people from underrepresented minorities who are achieving success in the U.S. science and engineering workforce. LSAMPs bring together public and private institutions that are Majority schools (many from the top tier of research institutions), historically Black colleges and universities (HBCUs), Hispanic serving institutions (HSIs), Tribal colleges, and community colleges.
Many ERCs have participated in the LSAMP program, either as members of their regional or state alliance, or by directly recruiting students from LSAMPs. Examples are, CPES, RMB, CISST, and CenSSIS (see sidebar). In 2005, for example, CPES developed a dedicated REU program specifically for LSAMP scholars.
The AGEP program is intended to achieve a significant increase in the number of students receiving doctoral degrees in the sciences, mathematics, engineering, and technology, with special emphasis on population groups underrepresented in these fields. In addition, since the lack of role models and mentors in the professoriate constitutes a significant barrier to producing minority graduates, NSF is particularly interested in increasing the number of minorities who will enter academic faculties in these disciplines. That is a major motivator for the AGEP program’s emphasis on funding institutions as well as students in ways that will lead to increases in doctoral degrees granted to minority students.
LSAMP Student’s Research in Cancer Detection In 2007, Nicolas Dedual was a student with the NSF-funded Gordon Center for Subsurface Sensing and Imaging Systems (Gordon-CenSSIS), an ERC based at Northeastern University (NU), and a participant in NSF’s LSAMP program. He worked with professors David Kaeli and George Chen to develop a four-dimensional visualization toolset. The toolset, called 4D-VIZ (four-dimensional visualization), was intended to help radiation oncologists track moving tumors in the body by allowing the user to view three-dimensional computed tomography images and display the images in a time sequence (time is the fourth dimension). |
Several ERCs have participated in AGEP. The Particle Engineering Research Center (PERC), for example, was very active in both AGEP and LSAMP involvement through its proactive Education Director, Anne Donnelly (see her profile in section 7-E(a), “Model ERC Educators”). In the early 2000s, PERC provided support for the management of the NSF-UF AGEP program and several AGEP scholars, all African Americans, worked with PERC faculty. Donnelly was for a number of years the co-PI and Director of the multi-institution SouthEast AGEP. Through Donnelly, the PERC also collaborated with the Florida-Georgia LSAMP (FGLSAMP) to increase the diversity of its undergraduate student population, not only through recruitment at the annual FGLSAMP EXPO, but also through collaboration with individual FGLSAMP institutions. The RMB, C-SOPS, and Synberc partnered with AGEP in various ways. For example, AGEP helped fund C-SOPS’ 2009 Winter Science and Engineering Forum, aimed at exciting undergraduates at predominantly undergraduate and minority-serving institutions in New Jersey about pursuing graduate studies in science and engineering.
Other NSF programs, such as the Centers of Research Excellence in Science and Technology (CREST), the Minority Postdoctoral Fellowship Program, and especially the Research Experiences for Undergraduates (REU) program have provided a stimulus and increased opportunities for women and underrepresented minorities to participate in all stages of the research process (see the sidebar for an example). All ERCs have had REU programs since it became a requirement in 1995. The ERC REU program was described in detail in Chapter 7, “Education and Outreach Programs”—see, for example, section 7-C(b).
______________________________________________________________________________________________________
CASE STUDY
Native American REU Student Becomes Academic Research Leader
Franklin Dollar, a member of the Pomo Indian tribe in California, was a 2012 Research Experiences for Undergraduates student at the Extreme Ultraviolet (EUV) ERC, based at Colorado State University. Now, as a faculty member at the University of California at Irvine (UCI), he is one of the faculty participant enablers in NSF’s STROBE Science and Technology Center (STC). (See Figure 10-20.)
Franklin Dollar grew up on a small Pomo reservation near Geyserville, California, with no running water, electricity, or internet access. At the time of writing, Dr. Dollar is an assistant professor of physics and astronomy at UCI and head of a lab studying ultra-high-intensity plasma physics. Joining UCI’s Department of Physics and Astronomy in Fall 2015, Dollar is only the second Native American physics professor in the nation’s top 50 schools.
An excellent high school science teacher sparked Franklin’s interest in science and led to his being accepted to UC Berkeley, where he majored in engineering physics. As an undergraduate student at Berkeley, Franklin worked closely with the Berkeley node of the EUV ERC, conducting research at the Lawrence Berkeley National Laboratory. His first paper as an undergrad acknowledged the EUV ERC.
After earning a Ph.D. in applied physics at the University of Michigan in 2012, Dollar became a postdoctoral scholar and research associate at the University of Colorado’s JILA institute, working with EUV ERC faculty members Margaret Murnane and Henry Kapteyn, who mentored him. There, he investigated X-ray source production with high-intensity lasers, a pursuit he has continued in his own lab at UCI. Murnane and Kapteyn also involved him with the design of the STROBE pathways program for Native American STEM students at Fort Lewis College in Durango, Colorado. Dollar also participated in drafting a proposal to procure NSF support for a new STC to explore how imaging modalities such as X-ray, optical, and electron microscopy can be improved and combined to help answer some of the most complex and challenging questions in medicine, chemistry, physics and other areas of science. The effort was successful; in fall 2016 the NSF announced a five-year grant of $24 million to fund the Science & Technology Center on Real-Time Functional Imaging (STROBE), with UCI as one of its nodes and Dollar as head of the work at UCI.
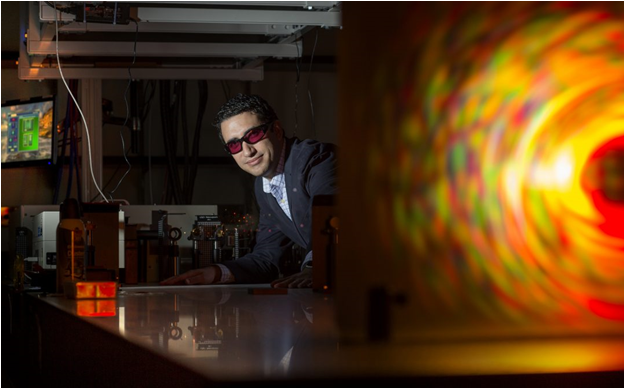
Figure 10-20: Franklin Dollar, assistant professor of physics and astronomy, grew up on an Indian reservation in a home with no electricity. Now he studies ultra-high-intensity laser-plasma interactions and works to increase diversity in scientific fields. (Credit: Steve Zylius/UCI) |
_________________________________________________________________________________________________________
v. Outreach Partners
In addition to their core partners, all ERCs since the late 1990s have had one or more—often several—“outreach partners.” The program announcement for the FY 1998 class of ERCS included among the specified key features the following:
“A dynamic, flexible program for outreach involving faculty and students from other universities and colleges to enhance the capacity of the ERC to fulfill its vision and develop connections with the community in its field”[81]
This feature included expanding not only the research but also the education mission to include outreach to non-partner universities and colleges and pre-college teachers and students. Much of the impetus for outreach was to reach both faculty and students in populations underrepresented in engineering, in order to engage a broader pool of participants in U.S. engineering and improve the diversity of the workforce. In some cases, even core partners were Historically Black Colleges and Universities (HBCUs) and Minority-serving Institutions (MSIs). Figure 10-21 depicts by name the “diversity partners” that were core partners, along with the locations of the outreach partners, of the 17 ERCs that were current in FY 2014.
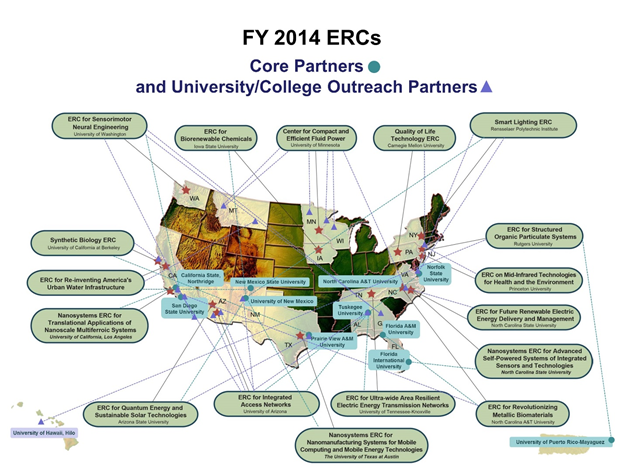
Figure 10-21: Diversity/Outreach Partners of FY 2014 ERCs
A number of these institutions were partners of more than one ERC. Most notably, the University of Puerto Rico at Mayaguez has been a core partner or outreach partner of seven ERCs since the late 1990s, in addition to providing REU students to many more ERCs. Overall, this university has had at least as much involvement with the ERC Program as any other U.S. institution. Several other institutions—especially in California, New Mexico, Texas, and Florida—have provided a strong connection for ERCs with Hispanic populations as core partners. A number of community colleges in western and midwestern states have provided ERCs with a connection to Native American students. One HBCU, North Carolina A&T University, has served as a lead institution (for the Revolutionizing Metallic Biomaterials [RMB] ERC).
10-D Conclusions
Looking back over the last 30 years, through the support of 71 ERCs, the Program did achieve a significant impact on the culture of engineering research and education on the lead, partner, and outreach engineering schools involved in these ERCs. The original call for the initiation of the Program in 1984 by the National Academy of Engineering was a forcing function for change at a time when the U.S. was under significant competitive challenges by Europe and Japan. There was a strong impact not only upon those engineering schools that succeeded in receiving an ERC award but also on those that competed unsuccessfully. The ERC Program was not alone in its response to these challenges, as the semiconductor industry formed the Semiconductor Research Corporation in 1984 to address the research needed to advance that technology and explore new ways to invest in academic engineering and science programs. The response also stimulated the ASEE and the ABET to promote a broader scope for engineering education that included industrial applications.
The cultural impacts of ERCs on the integration of disciplines in team-based research, the extension of engineering research to include strategic planning and engineered systems testbeds, and the integration of new findings from this research into new or revised curricula, as well as the creation of an engineering workforce better prepared for success in industry were the foundation for a wide range of impacts on these engineering schools. These features were also sustained in 82 percent of the graduated ERCs by 2010.
The 2001 Ailes study points out that “The interdisciplinary and collaborative nature of ERC research and education runs counter to traditional notions of individual faculty research results published in a well-defined set of disciplinary-based journals. Hosting an ERC thus required a university to consider and often to adjust its norms and policies for promotion and tenure. Deans, department heads, and ERC directors often reported having to “educate” P&T (Promotion & Tenure) committees about the emergence of collaborative research and publication. Usually a balance was struck, often informally, between a threshold of departmental work and a commitment to ERC research, although some universities did formal restatements of P&T requirements. ERC Directors are often influential in the P&T process, and while a few junior faculty were reported to have been discouraged from participating, few ERC participants failed to attain tenure. In many cases, the ERC participation was perceived as an advantage.”[82]
These impacts didn’t come easily; the lessons learned include:
- Rewarding risk taking at the intersection of disciplines
- Promoting interdisciplinary research through tenure and promotion practices
- Creating seed grants for young faculty to engage in cross/interdisciplinary research
- Truly collaborative interdisciplinary research requires time devoted to building a shared vision; and focused collaboration to integrate methodologies, vocabularies, and analytic approaches if the vision for a new engineering system is to be achieved.
Over time, this culture of collaboration not only led to successful ERCs but enabled explorations in research that led to new interdisciplinary fields, like bioengineering, neural engineering, synthetic biology, interfacial engineering, reconfigurable manufacturing, etc.
The systems construct and the ERC three-plane strategic planning chart enabled many ERCs to achieve complex, interactive engineered systems testbeds previously unheard-of in academic engineering. Notable examples, among many, are the BMES-Argus II retinal implant, the CASA testbeds to improved tornado warning systems, and the Rutgers continuous pharmaceutical manufacturing system, with its impact on FDA pharmaceutical manufacturing guidelines.
A compelling argument for the value of the ERC Program’s research-to-innovation construct through the three-plane strategic planning framework comes from the analysis of innovation and the ERC construct in a book authored by Steven Currall and his colleagues. They credit the framework as a tool to guide ERC leaders on how to move inventions derived from fundamental research toward proof-of-concept—technical demonstrations providing evidence that abstract ideas can have commercial potential. This is the foundation for their view of ERCs as vehicles for “organized innovation.”[83]
The impact of ERCs on engineering education was significant in their partner engineering schools and many of these innovations served as models for other engineering schools, such as the bioengineering degree programs at MIT and the reforms of electrical engineering education initiated by CMU. ERCs were also pioneers in forming partnerships with pre-college institutions and their teachers to bring engineering concepts and experiences to pre-college students. It must be recognized that while NSF expected ERCs to impact engineering curricula, none of that would have been possible without the enthusiastic support of a broad base of faculty and engineering and university administrators. Each ERC was successful in creating a research and education culture that effectively prepared engineering graduates for success in industry. Many became leaders in innovation throughout established firms or as CEOs of their own firms.
Underlying all these innovations in research and many of the innovations in engineering education were strong and interactive partnerships with industrial supporters and collaborators from a broad range of firms across the U.S. These partnerships would not have been possible without the changing culture of engineering research and education enabled by countless engineering administrators over the 30+ years since the Program began. In fact, two awarded ERCs were directly stimulated by industry groups representing two fields, microelectronics and fluid power.
ERCs and NSF led universities to develop
a more inclusive culture that engaged women and underrepresented minority
students in engineering at rates higher than the national average. This was
achieved through successive requirements and expectations that culminated in
strategic diversity plans developed with the departments and schools involved
in the ERCs.
[1] National Academy of Engineering (1984). Guidelines for Engineering Research Centers: A Report to the National Science Foundation, p. 14. Washington, DC: National Academy Press. [https://doi.org/10.17226/19472]
[2] Lewis, Courtland S. and James E. Williams, Jr. (2010). Post-Graduation Status of National Science Foundation Engineering Research Centers, a Report of a Survey of Graduated ERCs. Melbourne, FL: SciTech Communications, LLC., p. 1.
[3] Data provided to authors by ICF, with permission, 8/14/2019.
[4] ICF, formerly known as ICF International (ICFI); and from 2002 to 2009 by the QRC Division of Macro International, which was acquired by ICFI in 2009.
[5] ICF was replaced as the ERC Program’s data contractor in May 2019 by Creative Business Solutions, Inc. (CBS).
[6] Ailes, Catherine P., Irvin Feller, and H. Robert Coward (2001). The Impact of Engineering Research Centers on Institutional and Cultural Change in Participating Universities. Washington, D.C.: Stanford Research Institute, Science and Technology Program, p. 3.
[7] Currall, Steven C., Toby E. Stuart, Sara Jansen Perry, and Emily M. Hunter (2005). Engineering Innovation: Strategic Planning in National Science Foundation-Funded Engineering Research Centers, Report to the National Science Foundation. NSF Award Number: EEC-0345195, p. 6. Unpublished study available at http://erc-assoc.org/sites/default/files/topics/SCurrall_Final_Report_9-13-07.pdf
[8] Ailes et al. (2001), op. cit., p. 10.
[9] Ibid., p. vii.
[10] Ibid., pp. 37-38.
[11] Ibid., p. 64.
[12] Ibid., p. 15.
[13] See Section 10-A(a)i and especially Chapter 3 for more detailed definition of the differences between Gen-1 (1985-1990), Gen-2 (1994-2003), and the later Gen-3 (2008-2017) ERCs.
[14] Bill Costerton, Biofilm ERC, Montana State University; Dan Edie, CAEFF, Clemson University; Linda Griffith, MIT BPEC; Tom Harris, VaNTH, Vanderbilt University; Mark Humayun, BMES, University of Southern California; Margaret Murnane, EUV ERC, University of Colorado, Boulder; Brij Moudgil, PERC, University of Florida; Ulrich Neuman, IMSC, University of Southern California; Pietro Perona, CNSE ERC, Caltech; Jorge Rocca, EUV ERC, Colorado State University; Michael Silevitch, CenSSIS, Northeastern University; Bob Nerem, GTEC, GA Tech; Buddy Ratner, UWEB, University of Washington; Farhang Shadman, CEBSM SRC/ERC, University of Arizona; Bala Subramaniam, CEBC, University of Kansas-Lawrence; Ken Wise, WIMS ERC, University of Michigan; Mary Harper, Purdue University; Diana Rhoten, Social Science Research Council.
[15] Preston, Lynn (2004). “Mentoring Young Faculty to Success: Rewarding and Encouraging Involvement in Cross-Disciplinary Research.” Plenary Address to the ASEE Engineering Research Council.
[16] Ibid., slide 5.
[17] Ibid., slide 13.
[18] Ibid., slide 14.
[19] McLaughlin, David, V. Chandrasekar, Michael Zink, and Brenda Phillips (2014). CASA Engineering Research Center for Collaborative Adaptive Sensing of the Atmosphere, Final Report. Amherst, MA: University of Massachusetts-Amherst. P. 105.
[20] https://www.accessdata.fda.gov/scripts/cdrh/cfdocs/cfhde/hde.cfm?id=H110002
[21] See Chapter 3, Section 3-C for a detailed description of Gen-3 ERCs.
[22] Ailes et al. (2001), op. cit., p. 6.
[23] Ibid., p.13.
[24] Ibid., p. 80.
[25] Ibid., p. 23.
[26] Ibid., p. 32.
[27] Currall, Steven C., Ed Frauenheim, Sara Jansen Perry, and Emily M. Hunter (2014). Organized Innovation, A Blueprint for Renewing America’s Prosperity. New York, NY: Oxford University Press. p. 74.
[28] Ibid., p. 89.
[29] NSF (1997). Program Announcement: Engineering Research Centers, Partnerships with Industry, and Academe for Next-generation Advances in Knowledge, Technology, and Education, 1998. Arlington, VA: National Science Foundation, Directorate for Engineering, NSF 98-146, p. 1.
[30] Ibid., p. 4.
[31] McLaughlin, David, et al. (2014), op. cit., pg. 17.
[32] Wise, Kensall D. and Yogesh B. Gianchandani (2010). Summative Review Final Report, 2010, The ERC for Wireless Integrated MicroSystems (WIMS). Ann Arbor, MI: The University of Michigan. P. 124. Used by permission from Kensall Wise, 10/15/2018.
[33] Prof. Ken Wise’s 60 years in engineering are summarized in an “ECE Bicentennial & Beyond Lecture Series” on YouTube, in which he describes his history, the history of microelectronics in industry and academe, and his work in pressure, flow, and uncooled-infrared sensors; integrated interface circuits; and implantable microsystems for cardiovascular, intraocular, and neural disorders. Wise was one of the early leaders establishing the field of integrated sensors, now known as MicroElectroMechanical Systems (MEMS). (Personal communication, November 28, 2017.” View lecture at: https://www.youtube.com/watch?v=sBaz9z5J7bY
[34] Wise and Gianchandani (2010), op. cit., p. 65.
[35] Ibid., p. 78.
[36] Ibid., p. 58.
[37] Ibid., p. 72-73.
[38] Ibid, p. 75-77.
[39] Ibid., p. 124.
[40] The C-SOPS material was developed through an interview of Fernando Muzzio, Director C-SOPS, Rutgers University conducted by Lynn Preston. July 2018.
[41] National Science and Technology Council (2016). Advanced Manufacturing: A Snapshot of Priority Technology Areas Across the Federal Government. Report of the Subcommittee for Advanced Manufacturing of the National Science and Technology Council. Executive Office of the President, Washington, DC, April 2016. https://www.manufacturing.gov/sites/default/files/2018-01/nstc_sam_technology_areas_snapshot.pdf
[42] Ailes et al. (2001), op. cit.., p. 6.
[43] Ibid., p. 108.
[44] Currall et al. (2005), op. cit., p. 23.
[45] Ibid., p. 24.
[46] Ibid., p. 23.
[47] Ibid. pp. 24-25.
[48] Ibid., p. 24.
[49] Ibid., p. 25.
[50] Currall et al. (2014), op. cit., p. 50.
[51] Ailes et al. (2001), op. cit., p. 8-9.
[52] Ibid., pp. 91-92.
[53] http://www.gemstone.umd.edu/about/about-us.html
[54] http://news.mit.edu/2008/bioengineering-0602
[55] Ailes et al. (2001), op. cit., p. 83.
[56] Ibid., p. 22.
[57] Carnegie Mellon University(2000). Great Engineering Educators, Electrical and Computer Engineering. Brochure published by CMU, Pittsburgh, PA. p. 21. http://www.archive.ece.cmu.edu/about/_files/b007_ECE_great_enginrs.pdf
[58] McLaughlin, David et al. (2014), op. cit., pp. 33-34.
[59] https://www.cmu.edu/news/stories/archives/2012/april/april30_obituarycurtstone.html
[60] Siewiorek, Dan (2014). Quality of Life Technologies, Annual Report. Pittsburgh, PA: Carnegie Mellon University, Section 1.2.1.3, p.13.
[61] https://www.lib.ncsu.edu/specialcollections/centennialcampus/ehistory.html
[62] McKinney, Claude E. (1991). “Science & Technology: An Opportunity for Innovation in Community Design.” Presented at the International Seminar on Development Experiences of High Tech Industrial Parks, Nov. 21-22, 1991, Soowanbo, Korea, p.16. NCSU Archives, in https://www.lib.ncsu.edu/specialcollections/centennialcampus/ehistory.html
[63] https://projects.ncsu.edu/facilities/buildings/eng-grad.html
[64] https://www.engr.ncsu.edu/news/2018/05/21/college-mourns-loss-of-dean-emeritus-dr-nino-masnari/
[65] Section reviewed and approved by Jennifer Cox, Special Assistant to the Dean of Engineering, and other staff at NC State in October 2018.
[66] Fisher, John W. (1998). The Center for Advanced Technology for Large Structural Systems (ATLSS), Final Report. Bethlehem, PA: Lehigh University. March 31, 1998. p. 1.
[67] Ibid., p. 2.
[68] Ibid., p. 2.
[69] Ibid. p. 7.
[70] Ibid., p.3.
[71] Roesset, J.M. (1999). To the bottom of the sea. Scientific American issue on Extreme Engineering, 10(4), November 1999.
[72] Roesset, Jose M. (1999). Offshore Technology Research Center, a National Science Foundation Engineering Research Center (#8721512), Final Report. Texas A&M University. p. 2.
[73] See https://otrc.tamu.edu/otrc-wave-basin/
[74] After graduation from NSF ERC Program funding, GTEC was renamed the Regenerative Engineering and Medicine (REM) research center. It is still housed in the IBB.
[75] Husain, Iqbal (2018). Personal communication via email to Lynn Preston, 10/23/2018.
[76] See NSF (1995). Highlights of Engineering Research Centers Education Programs (NSF 95-56 (new). Arlington, VA: National Science Foundation.
[77] Personal communication with Lynn Preston, February 11, 2018. Use of quote approved by Brian Kelley via email.
[78] NSF (1995), op. cit., pp. 13-19.
[79] This list was developed by Matthew S. Croughan, a BPEC PhD graduate, who has kept up with the career pathways of his fellow BPEC graduates. It is used with his permission.
[80] So, Stephen (2011). “Sentinel Photonics and MIRTHE ERC: Starting up in an ERC.” Presented at the annual meeting of the ERC Program, December 1, 2011, Washington, DC.
[81] NSF (1997). Program Announcement: Engineering Research Centers—Partnerships with Industry and Academe for Next-Generation Advances in Knowledge, Technology and Education. Directorate for Engineering, National Science Foundation, NSF 97-5, p. 1.
[82] Ailes, op. cit. p. vii.
[83] Currall et al. (2014), op. cit., p. 50.